Quantum science: The quest for quantum information technology expands
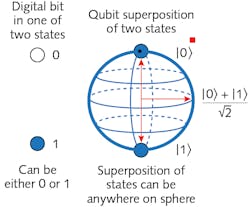
Exploratory research in quantum science has yielded promising advances towards new technologies: quantum sensors, quantum communications, and quantum computing. The United States recently joined the European Union, United Kingdom, China, Japan, Canada, and Australia in launching quantum initiatives to nurture those new technologies. A push by the National Photonics Institute, a joint effort of the Optical Society (OSA) and SPIE, led to bipartisan passage of a law establishing the National Quantum Initiative (NQI), signed into law on December 21, 2018. Plans call for the government to invest some $1.2 billion in quantum science over five years, with industry to invest additional money.
It’s an important and ambitious project, with government agencies and an industrial consortium hoping to transform the weirdness of quantum science into a practical powerhouse of quantum information technology. The first results are expected in a matter of years, but maturity may be decades away.
Government and industry teams
On the government side, the National Quantum Coordination Office, part of the White House Office of Science and Technology Policy, coordinates quantum efforts by the National Institute of Standards and Technology (NIST), the National Science Foundation (NSF), and the Department of Energy (DoE). Research grants flow to scientists through the three agencies. Military and intelligence agencies will continue their own separately funded quantum programs and coordinate with the NQI.
NIST provides start-up funding for the Quantum Economic Development Consortium (QED-C) to enable and grow the U.S. quantum industry. Over 100 U.S. companies have signed letters of intent to join, with formal support agreements to go out soon. QED-C’s emphasis on keeping industry and government in close contact with each other’s programs and needs is “unique in the world,” says its deputy director, Celia Merzbacher. Details are available at QED-C’s website: https://quantumconsortium.org.
Consortium members will pay dues and select what work to support. Much will be pre-competitive research to meet industry needs. Cryotechnology is a major need because quantum processors now operate close to absolute zero; QED-C has already developed a roadmap for it. On the other hand, Merzbacher expects the consortium will avoid funding projects of only academic interest or in hotly competitive fields.
Science first, technology follows
The big-picture goal is “science first,” says Michael Raymer, a quantum optics researcher at the University of Oregon (Eugene, OR), who coauthored a white paper proposing the NQI and co-led a lobbying effort to see it through. Scientific direction on the government side will come from the bottom up, with researchers writing proposals and peer reviewers selecting which to fund. The goal is to go beyond the use of quantum transitions in lasers and atomic clocks to “Quantum 2.0” applications that use quantum entanglement, including computing, communications, and sensing.
Conventional digital computing stores and processes information as digital bits that each have two states, 0 or 1, so its capacity to describe digital states increases linearly with the number of bits. Quantum computing stores and processes quantum objects called “qubits” (quantum bits) which can occupy superpositions of two states, 0 or 1. That subtle-seeming difference means that digital bits can have only one of two possible values, but qubits can have many more values because quantum processes can superpose their two quantum states in many ways as long as they maintain coherence, which as shown in Figure 1 means that the superposition can be anywhere on the surface of the sphere at right. Thus their capacity increases exponentially, so a line of 50 individual ion qubits trapped in a vacuum could represent up to 250 (about 1.125 × 1015) possible quantum states.
Quantum computers are not going to replace digital computers for balancing your checkbook or doing arithmetic. But quantum computers could excel at other types of processing that don’t come easily on digital computers because their quantum processing power increases exponentially with the number of qubits. That promises major advances in applications, including “simulating nuclear and high-energy physics; designing new chemicals, materials, and drugs; breaking common cryptographic codes; and performing more speculative tasks such as modeling, machine learning, pattern recognition, and optimizing hard logistical problems such as controlling the electric energy grid or traffic control systems,” Raymer and two colleagues wrote in the journal Science.1
Computing challenges
Quantum computers are much more vulnerable than digital ones to errors arising from noise and failure to maintain coherence. The “quality” of quantum bits depends on their isolation from the rest of the world needed to avoid overwhelming the signal with noise. So far, many groups have demonstrated qubits and simple types of quantum computing. In October 2019, Google claimed it had achieved “quantum supremacy” (solving a problem no classical digital computer could solve) with an array of 53 tunable qubits.2
That claim has been questioned and big challenges remain, yet most researchers believe so-called supremacy will be reached as the number of qubits is scaled up and suitable, useful algorithms are demonstrated.
Many types of qubits have been demonstrated, and their performance differs widely. Many consider the most versatile to be semiconductor devices made by photolithography, but they must be cooled very close to absolute zero to maintain coherence. Google’s Sycamore system operated at 20 mK; other semiconductor qubits operated at up to 100 mK. In April 2020, two separate groups reported using spin resonance in silicon to make qubits operable above 1 K,3 which may significantly simplify cooling requirements. One at the University of New South Wales (Sydney, Australia) reported a record high temperature of 1.5 K. A second, at QuTech and the Technical University of Delft (Delft, Netherlands), reached 1.1 K.4
Atomic ions trapped in electrostatic traps and coupled by lasers also can be used as qubits. Many groups are working in the field, and the claims are diverse. IonQ (College Park, MD) claims on their website the record for largest array with 79 qubits, but does not list details.5 In 2018, Ben Lanyon of the Institute for Quantum Optics and Quantum Information (Vienna, Austria) and colleagues reported 20 qubits—then the largest fully controllable, entangled system.6 In March 2020, Honeywell (Charlotte, NC) claimed a record “quantum volume” benchmark of 64 using four ion-trapped qubits cooled to 12.6 K.7 Figure 2 shows the ion trap apparatus. Honeywell says its advantage was “having the highest-quality fully connected qubits with the lowest error rates.” The company plans a commercial version in later this year.8D-Wave Systems (Burnaby, BC, Canada) already sells a quantum computer with 2000 supercomputing qubits, but outside observers say the qubits have limited connectivity and very short coherent times, and have yet to demonstrate entanglement to everyone’s satisfaction.9 Xanadu (Toronto, ON, Canada) uses integrated photonics to make photonic qubits that generate squeezed light and use it for quantum calculations and quantum machine learning.10 PsiQuantum (Palo Alto, CA) is fabricating silicon photonic qubits in a conventional semiconductor lab, and says on its website “Error correction is at the center of everything we do.”11 It seems likely that each of these different platforms may find their own niches where their hardware is most suitable.
Quantum sensing
Development barriers are lowest for quantum sensing, so it is likely to be the first quantum technology to be widely deployed, says Chris Haimberger, technology manager of Toptica-USA (Farmington, NY). Indeed, NIST already has reported an amazingly precise systematic uncertainty of 9.4 × 10-19 with an atomic clock based on quantum-logic spectroscopy of a transition in aluminum-27 ions.12
Quantum atomic interferometers can sense gravity and acceleration for navigating without GPS or for geological exploration. Quantum sensing can functionally image molecules on a nanometer scale, assess strain within materials, and measure magnetic fields in, for example, living cells.
Lasers can excite atoms with the high precision needed to create excited Rydberg states, which are just below the ionization threshold, and very sensitive to electric or magnetic fields. “Most of the sensors are much more about very precise control of the system than entanglement,” says Haimberger. Rydberg Technologies (Ann Arbor, MI) is offering radio-frequency field probes serving as optical frequency trackers.
Quantum communications and the quantum Internet
Quantum communications will be needed to transport qubits for quantum computing and other applications. Photons will carry the quantum information through space, air or optical fibers, like they carry digital bits. Depending on the wavelength used, quantum transmitters and receivers might need to be kept very cold to preserve quantum states and entanglement.
Qubits stored in a quantum memory are stationary physical objects, but their quantum states can be transported to a distant memory by encoding them as photon properties such as polarization. That encoded information could be transported by first entangling two other photons and sending them to different locations. Once those photons reach their destinations, it is possible to “teleport” the quantum state of the memory-based qubit to the distant memory from one site to the other. The process is not teleportation in the science-fiction sense of moving an object or a person through space. (It also is not instant, because it requires sending a subsidiary classical bit, which takes time.) Instead, it transports information on the quantum states, which can be used for remote quantum computing or other purposes.
Quantum state teleportation has stringent requirements, but it helps realize the potential of quantum computing and sensing. Encoding qubits as entangled photons would allow sharing their states to distribute quantum-computing tasks between locations. Quantum entanglement also offers a physical guarantee of security because it allows detection of eavesdropping. That enables secure distribution of a quantum key, which recipients could use to securely encrypt and decrypt a message. Another intriguing possibility is “blind” or private quantum computing, in which a person at one end of a quantum link could instruct a quantum computer on the other end to perform a quantum operation, then transmit the result back to the original source. Thanks to the security of entanglement, the results could be transmitted back to the original sender without anyone at the other end knowing the results.
Entangled photons can be transmitted about a hundred kilometers through optical fibers before the signal becomes too weak to detect reliably. They can travel much farther through free space. In 2017, a group led by Jian-Wei Pan, a physicist at the University of Science and Technology of China (Shanghai) stretched transmission of entangled photons to 1200 km by sending them from China’s Micius satellite through free space to a pair of ground stations, as shown in Figure 3.13 Too few photons got through for practical use, but the test helped stimulate U.S. support for the NQI.This summer, DoE will test transmission of entangled photons through 50 km of fiber linking its Argonne and Fermi National Laboratories in Illinois as a first step toward a “quantum Internet” linking its labs. DoE is working with NASA on plans for satellite-based tests using a technique called “entanglement swapping” using very precisely timed pulses of entangled photon states.14 One or two satellites would be based in low- or medium-Earth orbit, so signals could sweep across continents to connect with many locations.
Quantum optical signals decay with distance because of decoherence as well as attenuation, so maximum transmission distance is limited without quantum repeaters. Conventional optical amplifiers will not suffice because stimulated emission cannot reliably clone qubits, as spontaneous noise photons are always added at random. What’s needed to make a quantum repeater is a way to teleport qubits from one end to the other in order to retain entanglement. It’s a big challenge, but many concepts are under investigation. One example is using a cluster of about 10 photons to encode a qubit, and when one photon is lost, intercept the remaining ones, detect what was lost, and restore a copy of it. The idea is to be able to restore the missing photon without knowing the original quantum state it represented, but Raymer says it is still far from full implementation.
Plans and progress
As it emerges from startup mode, the QED Consortium is focusing on identifying quantum-enabling technologies and what developers need from them. They already have looked at cryogenics, a crucial field because most quantum devices operate near absolute zero, and the special cooling equipment is large and costly. Merzbacher says they are planning a workshop on quantum-enabling laser technologies.
One QED-C study group is studying how to report progress in meaningful ways, so the user community can understand the system capabilities. “In quantum computing, people like to report the number of qubits, but that’s not a good measure of performance because there are issues of quality as well as quantity,” says Merzbacher. It’s a problem that is painfully evident when reading recent press releases. Other issues include developing a workforce skilled in quantum technology, and coordinating information flow among industry, government, and academia. The Optical Industry Development Association (OIDA) is working on a roadmap of photonics needed to develop quantum technology. It all adds up to a big job.
Progress is encouraging, but quantum technology is still in its early stage. “In two to five years we will have quantum computers that do something useful,” says Mark Tolbert, CEO of TOPTICA-USA, who is actively involved with QED-C and the NQI. Honeywell, IonQ, Google, and others have demonstrated devices, but he says they are “still trying to figure out what they can do with them.”
The mainstream view, Tolbert says, is that more general use of quantum computers is 10 to 20 years away. He says quantum sensing is likely to come first, followed by quantum communications, where he has seen been major advances in the past six months. Raymer thinks the full suite of technology may take 20 to 30 years to mature.
Look back 20 to 30 years, and you can see the birth of the Internet and a time when 56 kbit/s modems seemed fast and we thought a megabit to the home would be blindingly fast. Look forward that far, and you can expect another transformation of information technology.
REFERENCES
1. C. Monroe, M. G. Raymer, and J. Taylor, Science, 364, 440–442 (May 3, 2019); doi:10.1126/science.aax0578.
2. F. Arute et al., Nature, 574, 505–510 (2019); https://bit.ly/HechtRef2.
3. C. H. Yang et al., Nature, 580, 350–354 (Apr. 16, 2020); https://bit.ly/HechtRef3.
4. L. Petit et al., Nature, 580, 355–359 (Apr. 16, 2020); https://go.nature.com/2zTwrM3.
5. See https://bit.ly/HechtRef5.
6. B. Lanyon et al., Phys. Rev. X, 8, 021012 (Apr. 10, 2018); https://bit.ly/HechtRef6.
7. See https://bit.ly/HechtRef7.
8. See https://bit.ly/HechtRef8.
9. C. Bruzewicz, J. Chiaverini, R. McConnell, and J. M. Sage, Appl. Phys. Rev., 6, 021314 (2019).
10. See https://bit.ly/HechtRef10.
11. See https://bit.ly/HechtRef11.
12. S. M. Brewer et al., Phys. Rev. Lett., 123, 033201 (Jul. 15, 2019); https://bit.ly/HechtRef12.
13. J. Yin et al., Science, 356, 6343, 1140–1144 (Jun. 16, 2017); doi:10.1126/science.aan3211.
14. D. Oberhaus, “NASA’s plan to turn the ISS into a Quantum Laser Lab,” Technology Review (Apr. 22, 2020); https://bit.ly/HechtRef14.
About the Author
Jeff Hecht
Contributing Editor
Jeff Hecht is a regular contributing editor to Laser Focus World and has been covering the laser industry for 35 years. A prolific book author, Jeff's published works include “Understanding Fiber Optics,” “Understanding Lasers,” “The Laser Guidebook,” and “Beam Weapons: The Next Arms Race.” He also has written books on the histories of lasers and fiber optics, including “City of Light: The Story of Fiber Optics,” and “Beam: The Race to Make the Laser.” Find out more at jeffhecht.com.