PHOTONIC FRONTIERS: HIGH-EFFICIENCY PHOTOVOLTAICS: Photovoltaics takes small steps on journey to greater efficiency
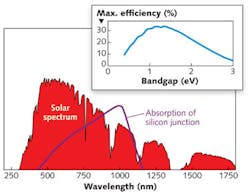
Progress in photovoltaics comes in small steps. On Oct. 15, 2012, Solar Junction (San Jose, CA) announced that its multijunction solar cells had converted 44% of incident highly concentrated sunlight into electrical energy, breaking the 43.5% record the company had set in April 2011. The National Renewable Energy Laboratory (NREL; Golden, CO) verified both measurements.
That may seem at best an incremental gain compared to the Moore's law doubling of capacity in technologies such as integrated electronics or fiber-optic transmission. But it represents real progress in power conversion, where efficiency is constrained by a fundamental limit on how much energy a p-n junction can collect, recognized half a century ago by William Shockley, best known as a co-inventor of the transistor.1
The Shockley-Queisser limit on efficiency
Shockley and Hans Queisser recognized that a single junction can absorb only a fraction of the broad blackbody spectrum of sunlight reaching the ground. The junction absorbs only photons with energy above the bandgap, and if photons have more than the energy needed to excite a valence electron into the conduction band, that extra energy is lost rather than converted into electricity, as shown in Fig. 1. This limits energy efficiency to a peak value that depends on the bandgap, as shown in the inset. For silicon (Si) with a 1.1 eV bandgap, which is plotted in Fig. 1, that theoretical limit is 33.7%.
However, that limit applies only to a single junction, and it's possible to stack two or more junctions with different bandgaps to make a multijunction solar cell with higher efficiency. Solar Junction stacked three junctions, spaced across the spectrum to extract 44% of the energy in concentrated sunlight, which marks the cutting edge of solar-cell efficiency. Optical losses and other practical issues keep that a long way from the theoretical limit of 86% for an infinite stack of lossless junctions with concentrated sunlight.2
High efficiency thus requires multijunction cells, although their cost and complexity limit their applications to powering spacecraft and generating power on the ground from concentrated sunlight, which also enhances efficiency.
Multijunction solar cells
Multijunction cells divide sunlight among a series of single junctions that absorb different slices of the spectrum. Typically layers are stacked on top of each other, with light incident on the largest-bandgap layer, which transmits longer wavelengths to smaller-bandgap layers underneath.
Figure 2 shows the structure and absorption of a conventional three-junction cell, with a bottom germanium (Ge) junction on a Ge substrate, covered by layers of indium gallium arsenide (InGaAs) and indium gallium phosphide (InGaP). The top InGaP layer absorbs photons with energies of at least 1.88 eV, transmitting lower-energy photons to the InGaAs with a 1.4 eV bandgap. Wavelengths longer than 900 nm are transmitted to the 0.7 eV Ge layer, which absorbs out to 1800 nm, where sunlight reaching the ground drops to near zero.Conversion efficiency is 37–40% when sunlight is concentrated to about 500× normal intensity. Electrically in series, the three junctions together have open-circuit voltage of 3.2 V, with current limited by the lowest-current junction. Spectrolab has reached 41.6% efficiency in the lab.3 "That's an amazing achievement, showing that doing things perfectly can get you a long way," says Daniel Friedman, manager of the III-V multijunction photovoltaics group at NREL. But that's about the limit. With production cells at 39–39.5% efficiency under concentrated sunlight, Spectrolab developers wrote this year, "there appears to be little opportunity for further efficiency gain with this three-junction technology."4
The limit comes from the Ge layer, which nicely matches the lattice spacing of low-indium 1.4 eV InGaAs but has a bandgap much lower than the 1 eV that is the theoretical ideal for a three-layer cell. The challenge is that 1 eV ternary III-V semiconductors don't lattice-match GaAs or InGaP. Overcoming that problem requires either strain-accommodation structures or exotic III-V compounds.
Strained-layer photovoltaics
Adding 30% In to GaAs reduces its bandgap to 1 eV but also increases its lattice constant too much to allow defect-free growth of low-indium InGaAs or InGaP. Strained-layer structures allow the growth of InGaAs pump diodes on GaAs substrates, but those strained layers are near the top. For multijunction photovoltaics, the 1 eV layer must be under the higher-bandgap layers, and that's a problem because all additional layers must accommodate the strain.
"If you are going to grow a layer which is strained, or has a different lattice constant than you start with, it's best to leave that layer as late as possible in the growth of a structure," says Friedman. To overcome that problem, his group at NREL developed the inverted metamorphic structure, in which the top InGaP layer is grown first on a matched substrate such as Ge or GaAs, followed by lightly doped GaAs, and a 1 eV In0.3Ga0.7As layer. Then the epitaxial layers are removed and flipped so the InGaP layer is on top. In May 2012, Sharp Corp. (Osaka, Japan) reported 43.5% efficiency at 306 suns using that technique. Spire Semiconductor (Hudson, NH) earlier reached 42.3% efficiency at 406 suns by first growing a lattice-mismatched 0.94 eV InGaAs junction on GaAs, then flipping the wafer and growing lattice-matched GaAs and InGaP cells on the other side.5
Dilute nitrides at Solar Junction
Solar Junction's record-setting photovoltaics replace Ge with a GaAs junction containing 8% In and 2.8% N added to adjust the bandgap to 1 eV and match lattice spacing to GaAs. They added antimony as a surfactant. Adjusting the indium and nitrogen concentrations in the dilute nitride can vary bandgap between 1.4 and 0.8 eV. Metal-organic vapor-phase epitaxy (MOVPE), standard for other solar cells, cannot grow high-quality dilute nitrides, but Solar Junction succeeded with a process based on molecular-beam epitaxy (MBE), which can grow delicate thin-layer structures.
In early 2011, Solar Junction used this structure to reach a record 43.5% efficiency at 418 suns. Since then the company has targeted 1000-sun intensities sought by customers, which heat the cells to 60°–90°C. Now they have improved their processing to reach 44% efficiency at 1000 suns with cells from their production line.
"The cells are a lot better than they were a year and a half ago," explains Vijit Sabnis, vice president for technology at Solar Junction. One key improvement was reducing resistance of the electricity-collecting grid on top of the cell, which requires adding lines that reduce the light reaching the junctions.
"To grab that efficiency back, your overall material quality has to be better, producing more current with less light hitting the cell," says Sabnis. "Although we only increased efficiency by half a percent, the cell is operating much better." Like other semiconductor devices, the 44% efficiency drops with increasing temperature, but Sabnis says it remains above 40% at 90°. He doesn't think that's the limit, saying, "I wouldn't be surprised if we could get another 0.5–1% out of triple junctions."
More junctions and opportunities
Adding more junctions could further increase efficiency. Substituting a 1 eV material for 0.7 eV Ge leaves wavelengths from 1300 to 1800 nm unused, so Solar Junction is now putting its three-layer dilute nitride cell on top of a Ge layer to collect energy at the longer wavelengths, as shown in Fig. 3. "It will take a couple years to get there," Sabnis says, and he hopes to reach 47–48% efficiency with highly concentrated sunlight.Further efficiency improvements would come from cutting the spectrum into thinner slices, so less energy is lost from photons above the bandgap energy. To add a fifth layer, Sabnis envisions adding a second dilute nitride layer with a different bandgap energy. Adding aluminum to other junctions and further innovations such as high-transparency tunnel junctions between layers "are going to take us up to 50%," a goal he concedes will challenge engineers but does not require any fundamentally new science.
Converting half the input concentrated solar energy to electrical energy need not be the limit, Albert Polman of the FOM Institute AMOLF (Amsterdam, the Netherlands) and Harry Atwater of the California Institute of Technology (Pasadena, CA) wrote recently in Nature Materials.6 "Recent advances in the control of light at the nanometer and micrometer length scales, coupled with emerging materials fabrication approaches" should allow multijunction cells to reach 50–70% efficiency, they write. "The key challenge now, and one that has great potential, is to better engineer the flow of light inside a solar cell."
That bold vision won't make Moore's law apply to photovoltaic efficiency, but it could open the way to progress in larger steps than fractions of a percent.
REFERENCES
1. W. Shockley and H.J. Queisser, "Detailed Balance Limit of Efficiency of p-n Junction Solar Cells," J.Appl. Phys., 32, 510 (1961); http://dx.doi.org/10.1063/1.1736034.
2. A. De Vos, "Detailed balance limit of the efficiency of tandem solar cells," J. Phys. D:Appl. Phys., 13, 839 (1980); http://dx.doi.org/10.1088/0022-3727/13/5/018.
3. R.R. King et al., "Solar cell generations over 40% efficiency," pres. at 26th European Photovoltaic Energy Conference, Hamburg, Germany, Prog. Photovolt: Res. Appl. (2012); doi:10.1002/pip.1255.
4. R.K. Jones et al., "Evolution of Multijunction Solar Cell Technology for Concentrating Photovoltaics," Japanese J.Appl. Phys., 51, 10ND01 (2012); http://dx.doi.org/10.1143/JJAP.51.10ND01.
5. P. Chiu et al., "42.3% Efficient InGaP/GaAs/InGaAs concentrators using bifacial epigrowth," pres. at IEEE Photovoltaic Specialists Conference (PVSC 2011); doi:10.1109/PVSC.2011.6186067.
6. A. Polman and H.A. Atwater, "Photonic design principles for ultrahigh-efficiency photovoltaics," Nat. Mat., 11, 174–177 (March 2012).
About the Author
Jeff Hecht
Contributing Editor
Jeff Hecht is a regular contributing editor to Laser Focus World and has been covering the laser industry for 35 years. A prolific book author, Jeff's published works include “Understanding Fiber Optics,” “Understanding Lasers,” “The Laser Guidebook,” and “Beam Weapons: The Next Arms Race.” He also has written books on the histories of lasers and fiber optics, including “City of Light: The Story of Fiber Optics,” and “Beam: The Race to Make the Laser.” Find out more at jeffhecht.com.