PHOTONIC FRONTIERS: OPTICAL ANTENNAS: Optical antennas concentrate light and direct beams
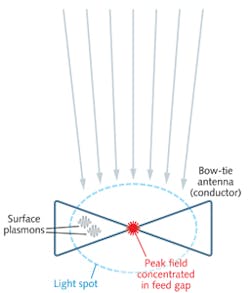
Radio antennas played a crucial role in electronics, converting electric signals into radio waves at transmitters and converting the waves back into electrical signals at receivers. Now antennas are being shrunk orders of magnitude to nanoscale for photonic applications.
Optical antennas rely on the same principles of electromagnetic theory as radio antennas. Oscillating electric charges collectively radiate electromagnetic waves in a pattern that depends on the antenna structure and the oscillation frequency. Receiving antennas absorb incoming radiation, converting it into heat and oscillating charges. But although the principles are the same, the frequencies are hundreds of thousands times higher, so the workings of optical antennas differ in important ways from those of radio antennas.
So far, most optical antenna work has focused on proof-of-principle demonstrations, says Palash Bharadwaj of the Swiss Federal Institute of Technology (Zurich, Switzerland). The results have been promising, but technological applications have been slowed by the need to make wavelength-scale components accurate to within 10 nm.1
Optical antenna fundamentals
Electromagnetic theory scales simply with wavelength, and more than half a century ago Richard Feynman envisioned nanoscale antennas when he told the American Physical Society, "there's plenty of room at the bottom." He wondered if arrays of little antennas might stick up from circuits measuring 100 to 1000 nm. "Is it possible, for example, to emit light from a whole set of antennas, like we emit radio waves from an organized set of antennas to beam radio programs to Europe? The same thing would be to beam the light out in a definite direction with a very high intensity," he said.2
However, Feynman recognized that material response to light frequencies differs greatly from that to radio waves. Electrical currents in conductors can easily vary at the gigahertz rates needed for radio antennas, but not at the hundreds of terahertz of light waves. The oscillating charges in optical antennas are surface plasmons, which move very fast, but conductivity is low at optical frequencies. Surface plasmons require much higher electron mobility than radio antennas, so few conductors can serve as optical antennas, notably silver and gold.
Importantly, optical excitation of a subwavelength antenna can produce surface plasmons much smaller than the light wave, as shown in Fig. 1 for a bow-tie antenna. "It's cool because it allows you to make up for the mismatch between the size of the photon, a wavelength of visible light, and true nanoscale materials," says James Schuck, director of the Molecular Foundry at the Lawrence Berkeley Laboratory (Berkeley, CA). Optical antennas also are being explored for applications including beam steering and direction.
Spectroscopy, heating, and high harmonic generation
Many important potential applications have energy concentration in an optical antenna. "Shine a laser onto a pointed gold or silver tip in close proximity to something you want to study, and the strongly enhanced field at the apex of the tip leads to enhanced Raman response" for tip-enhanced spectroscopy, says Bharadwaj. The tip may not look like a traditional antenna, but it concentrates the propagating light energy into a subwavelength region, so it can map defects in carbon nanotubes with resolution of tens of nanometers. The same technique can improve the sensitivity of spectroscopic bioassays.
Visible light is a particularly valuable probe because it gathers information on chemical bonds and molecular structure, says Schuck. "If you want to understand the structure, the easiest way is to probe with optical photons." With nanoantennas, light can achieve resolution of 10 nm—the size of the "feed gap" between the two halves of a dipole-type antenna, where energy absorbed by the whole antenna is concentrated.3 Figure 2 shows how energy is concentrated in a campanile-type antenna, shown in the inset.Concentrating light in such a gap also can produce the high electric field intensities needed for harmonic generation. Researchers at the Korea Advanced Institute of Science and Technology (Daejeon, South Korea) used that approach to concentrate 10 fs pulses with peak power of 100 kW by a factor of 100, reaching 1013 W/km2. That allowed them to produce the 17th harmonic at 47 nm in an argon jet aimed at the gap.4 This could allow compact sources to generate high harmonics.
Nanoantennas also can concentrate light energy to heat tiny regions. When Naomi Halas's group at Rice University (Houston, TX) illuminated gold nanoparticles in water with a resonant wavelength, the particles absorbed so much heat that it vaporized the surrounding water, leaving the nanoparticles in a steam bubble.5 This avoids the need to heat the whole fluid to boiling, and potentially could lead to clinical applications such as destroying tumor cells that had absorbed nanoparticles.
Antennas and light detection
Optical antennas also can enhance light detection when they are attached to semiconductors. Mark Brongersma's group at Stanford University (Stanford, CA) increased the photoresponse of a germanium nanowire more than a factor of 25 by adding contacts at each end, one ohmic and one Schottky (metal-semiconductor), creating a resonant nanoantenna with a response above 100 GHz. Another benefit of the design is that changing the diameter of the nanowire shifted the resonant peak from 1300 to 1530 nm, attractive for communication applications.6
Halas's group took a different approach, capturing the energy produced when surface plasmons in an optical antenna decay to hot electron-hole pairs. They fabricated an array of 300 rectangular gold nanorods on the surface of n-doped silicon, forming a Schottky barrier between the two materials. Hot electrons generated in the gold nanoantenna had enough energy to cross the Schottky barrier and produce a photocurrent in the silicon. The wavelength response depends on the nanoantenna resonance and extends beyond the semiconductor bandgap. They reported that quantum efficiency was limited to about 0.01% in their laboratory devices, but suggested that adding a 1 V reverse bias and improving fabrication could raise quantum efficiency to nearly 2%. As a silicon-based device with response extending into the InGaAs detector range, it could be attractive for silicon photonics or silicon infrared imaging systems.7
Transmitters, beam shaping, and steering
In principle, optical antennas should improve the efficiency of inefficient emitters such as organic LEDs (OLEDs). However, more success has been reported so far in developing optical analogs of phased arrays of radio antennas to shape and steer emitted beams.
One approach is creating a metasurface, a flat array of plasmonic antennas with subwavelength spacing that change the phase and polarization of scattered light, creating beams with controllable direction, polarization, and orbital angular momentum. In a review paper, Nanfang Yu of Columbia University (New York, NY) and colleagues cite demonstrations of "phased arrays that beam light into arbitrary directions, flat lenses that create converging spherical waves, planar wave plates that operate over ultrabroadband, and spiral phase masks that generate optical vortex beams."8 Yu earlier used subwavelength surface structures to narrow emission of a terahertz quantum cascade laser from 180° to 10°.9
Another approach is building an array of many tiny light-emitting optical antennas, with phases shifted between adjacent emitters. Passive arrays can project fixed images or beams in a constant direction; active phase-shifting of individual emitters allows beam steering. Active beam steering is preferred for many applications, but the elements could not be packed closely together using conventional optical couplers and phase shifters, which are tens to hundreds of wavelengths long.Michael Watts and colleagues at MIT overcame those limits by fabricating light-delivery waveguides and emitting antennas in silicon on complementary metal-oxide-semiconductor (CMOS) material. They fabricated a passive 64 × 64-element array in which a single waveguide running along one side of the array splits input light among secondary waveguides serving the rows, and each of those waveguides divides the light among antennas along one row. Figure 3 shows a part of this array. To make a steerable array with 8 × 8 elements, they added silicon electronic connections and applied heat to the waveguides to shift the phase actively using the thermo-optic effect.10
Outlook
Proof-of-principle demonstrations have yielded exciting results, but actual applications of optical antennas are few and far between. The ability to focus hot spots of energy onto 10 nm spots is attractive for optical imaging and for spectroscopic probes, says Schuck. Enhancing optical fields in small volumes is attractive for ultra-sensitive detection, such as spotting toxins before they reach dangerous concentrations. One long-term possibility is developing nanoantennas that could be concentrated into tumor cells, where they could selectively absorb enough energy to kill the cells. Both Schuck and Bharadwaj see heat-assisted magnetic recording for a new generation of ultra-high-density hard drives as a very promising application for optical antennas.
Many other applications may be possible in the long term, such as beam steering to switch optical signals on chips. As Feynman said, there is plenty of room at the bottom. But much work remains to turn those visions into practical realities.
REFERENCES
1. P. Bharadwaj, B. Deutsch, and L. Novotny, Adv. Opt. Photon., 1, 438–483 (2009); doi:10.1364/aop.1.000438.
2. R. Feynman, "There's plenty of room at the bottom," Eng. Sci., 23, 5, 22 (May 1960).
3. W. Bao et al., Science, 338, 1317 (2012); doi:10.1126/science.1227977.
4. S. Kim et al., Nature, 453, 757 (June 5, 2008); doi:10.1038/nature07012.
5. Z. Fang et al., Nano Lett., 13, 1736–1742 (2013).
6. L. Cao et al., Nano Lett., 10, 4, 1229–1233 (2010); doi:10.1021/nl9037278.
7. M. W. Knight et al., Science, 332, 702 (2011); doi:10.1126/science.1203056.
8. N. Yu et al., IEEE J. Select. Topics Quantum Electron., 19, 3, 4700423 (May/June 2013); doi:10.1109/jstqe.2013.2241399.
9. N. Yu et al., Nat. Mater., doi:10.1038/nmat2822 (Aug. 8, 2010).
10. J. Sun et al., Nature, 493, 195–199 (Jan. 10, 2013); doi:10.1038/nature11727.
About the Author
Jeff Hecht
Contributing Editor
Jeff Hecht is a regular contributing editor to Laser Focus World and has been covering the laser industry for 35 years. A prolific book author, Jeff's published works include “Understanding Fiber Optics,” “Understanding Lasers,” “The Laser Guidebook,” and “Beam Weapons: The Next Arms Race.” He also has written books on the histories of lasers and fiber optics, including “City of Light: The Story of Fiber Optics,” and “Beam: The Race to Make the Laser.” Find out more at jeffhecht.com.