ADVANCED APPLICATIONS: LASER INSTRUMENTS: Lasers explore beyond the microscopic world
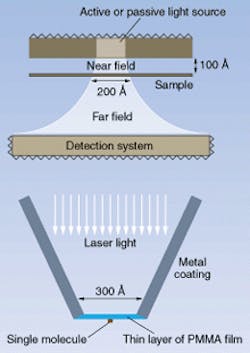
Measuring the expansion of a bone to a fraction of picometer, manipulating microscopic crystals, and probing the spectra of single molecules are some of the more-impressive recent achievements of laser-based instruments. Still, even as this class of equipment continues its precision probing of the ultrasmall world, it is also becoming a workhorse of environmental and pollution-monitoring applications.
Taking precision to extremes
One long-term challenge of instrumentation has been to measure lengths to increasing levels of precision under a widening variety of conditions. Laser techniques have long been at the forefront of this effort because of their unique processing capabilities. Today, it is possible to design economical laboratory devices that can measure lengths accurately to a few tenths of a picometer—a few thousandths of the diameter of an atom.
A recent example of such work is an instrument developed at the University of Pisa in Italy to carry out delicate measurements of the piezoelectric effect on bone.1 It has been long known that human and animal bones are piezoelectric and generate small electrical currents when stressed. These currents appear to signal bone cells when more calcium is needed to strengthen the bone. The Italian research team wanted to study the inverse piezoelectric effect, in which the electric field generates extremely small changes in bone length. With maximum acceptable fields of 1 kv/cm, piezoelectric displacements of only a few picometers were expected.
The Pisa researchers placed each of two bone samples from a cow against cantilever arms normally used for atomic force microscopes. A beam from a helium-neon (HeNe) laser was then split and bounced off gold mirrors on one face of the cantilevers. The beams traveled to two photodetector pairs covering part of the output beam. When a deflection from the cantilever moved the beam, the balance between the photodetector signals changed, producing a signal. The electric field was applied to only one sample, but vibration and thermal noise affected both paths equally so they could be subtracted, leaving only the piezoelectric expansion effect.
Because the cantilever arm is only 100 µm long and the photodetectors are placed 5 cm from the sample, the magnification of the displacement was five hundredfold. This, however, only brought the expected displacements into the 0.1-nm range or one ten-millionth of the laser spot diameter. The noise level for a single measurement was 0.65 nm of initial displacement or 0.03% of the spot diameter. With a sampling rate of 1 kHz, the average noise rapidly decreased to a few tenths of a picometer for averages exceeding 10,000 measurements.
Detecting single molecules
Two other interesting instrumentation-related developments of the past few years have involved the perfection of the near-field-scanning-optical microscope (NSOM) and near-field-scanning-optical spectroscope. In the near field of a small aperture, a light source illuminates only the area of the aperture, even if it is much smaller than a wavelength of light. While Edward Hutchinson theorized about the use of this effect for new types of microscopes as early as 1928, the technique was not put into practice until the 1980s. Only in recent years has the technology developed for other scanning techniques, such as atomic force microscopy, been fully applied to perfecting near-field optical microscopy.
In a NSOM, laser light funnels through a micropipette to an aperture typically 30-100 nm across. The light reflected from the illuminated area of a sample is then piped back up the probe to detectors. Recent research is attempting to improve the already high resolutions offered by the technique. Several laboratories have attempted to attach a single organic molecule to the tip of the NSOM probe.2 In one example, researchers attached a carbocyanine dye molecule to a thin film at the probe tip (see Fig. 1). Laser energy excited the molecule, causing it to emit a different wavelength of light. The single molecule then acted as the near-field probe, illuminating an area only a nanometer or so across. Applications of such single-molecule probes include detecting single molecules of substances, sequencing DNA, monitoring individual molecule reactivity, and detecting disease at an early stage.
Managing micromanipulation
Laser instruments are used not only to detect very small objects, but also to manipulate them. For example, optical tweezers—which use laser light emitted from very narrow probes to push around small objects—have become routine tools in the laboratory.
Optical forces exist because photons carry momentum, which generally is negligible but becomes significant for high light intensities and small objects. One new application of this technique involves controlled seeding of crystal growth. Researchers at the Institut Des Materiaux de Nantes (Nantes, France) have successfully picked up individual seed crystals about 25 µm across using laser levitation. Here, a weakly converging laser beam confines the seed in the transverse direction due to the gradient of laser intensity, but gently pushes the seed along the direction of the beam. Alternatively, a beam can be directed downward toward a seed resting on a surface so that it can gently push the seed using transverse gradient forces.
Dissecting the environment
Even as laser instruments in the laboratory have begun probing smaller samples, other traditional laser-based laboratory instruments have been moving out into the field to measure pollution in the environment. For example, lidar techniques, which have long been used to probe atmospheric pollution, are now being adapted to underwater monitoring, as well.
In work performed at the ENSSAT Center in Lannion, France, a frequency-doubled Nd:YAG laser is used to generate a fluorescence signal from particles suspended in water. An optical antenna consisting of a bundle of optical fibers picks up the return signal, which is then fed to the spectrometer. A cut-off filter at 580 nm prevents the strong, 532-nm reflected signal from entering the spectroscope. A CCD camera digitally records the spectroscopic output from each fiber, producing an image resolved in both space and wavelength.
Using a 25-Hz repetition rate and 6-ns pulses, the tomoscopic laser spectroscope can scan over a volume of water, with the delay time for the return signal indicating the distance to each volume of water. A complete three-dimensional spectroscopic survey results. It can then be used to detect both pollutants and vegetationsuch as by indicating chlorophyll concentration.
Laser-induced fluorescence spectroscopy is also moving into the field in all-solid-state instruments for analysis of key pollutants. Polycyclic aromatic hydrocarbons and mono-aromatics such as benzene and toluene are high priority for detection as they are highly carcinogenic. Last year, a group at the Laser-Laboratorium (Göttingen, Germany) reported the development of a compact battery-powered instrument that operates at 266 and 355 nm.3 The device feeds either wavelength through a fiber to a detection head, and the fluorescence signal comes back through the same fiber head (see Fig. 2). Time-resolved spectra of the resulting fluorescence are recorded and the data compared with known spectra to yield a quantitative analysis of each substance in the water.Even as laser instrumentation in the laboratory continues to measure at smaller and smaller scales, laser-based instruments that were primarily laboratory-based options are becoming more routine and ubiquitous in the field. These application-broadening trends are likely to continue in the future.
REFERENCES
- P. Gizdulich et al., Meas. Sci. Technol. 10, 232 (March 1999).
- W. Tang, Trends in Analytical Chemistry 17, 501 (Sept.1998)
- F. Lewtizka et al. (in press 1999).
About the Author
Eric J. Lerner
Contributing Editor, Laser Focus World
Eric J. Lerner is a contributing editor for Laser Focus World.