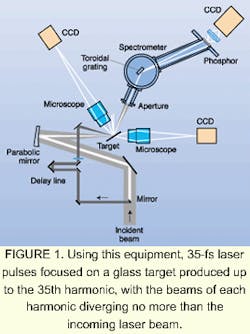
The production of femtosecond laser pulses—often with only a few oscillations and extremely high intensity—has become almost a common-place activity in hundreds of small and midsize research laboratories around the world. Relatively inexpensive compact tabletop laser systems are now generating shorter, more-intense pulses than those previously possible with equipment only at the largest government laboratories. Since 1987, when the current methods of compressing laser pulses were first developed, the maximum intensity of laser pulses—the concentration of power per unit area—has doubled every few months, with no limit yet in sight.
Ultrafast lasers now can deliver pulses shorter than 5 fs with only a couple of oscillations of light. Peak powers have exceeded 1300 TW, with intensity on targets of 1021 W/cm2. Such intense radiation can accelerate electrons to nearly the speed of light, generate pressures hundreds of times those at the center of the Earth, and create quite powerful magnetic fields.
In these extreme conditions, researchers can probe the behavior of matter at high energy and test theories ranging from astrophysics and general relativity to quantum mechanics. Such pulsing capability may even be the key to providing very compact particle accelerators or generating fusion energy.
What is perhaps equally exciting is that, like computer circuits, high-power lasers have shrunk dramatically in the last decade in both cost and size. Some tabletop lasers offer extremely high performance at a cost that is within the reach of some smaller laboratories at universities and hospitals. Experiments that previously might have been contemplated only at large facilities with millions of dollars can now be performed with small teams at dozens of facilities.
Chirped pulse amplification
The key to this rapid advance was the development in the late 1980s of chirped pulsed amplification. Prior to that, materials appeared to limit the intensity that laser pulses could reach. At intensities approaching gigawatts per square centimeter, the index of refraction of the gain material varies linearly with intensity, so that a beam that is more intense in the center produces a higher index of refraction at the center, creating a lensing effect and destroying beam quality. Because this limits intensity, short-pulse production requires using materials with low energy density such as dyes and excimers with energy densities of only millijoules per square centimeter. This in turn meant that lasers with any respectable energy output had to be large and expensive.
Originally, chirped pulse amplification was developed for radar, but it translated well into the optical regime. With amplification, a laser produces a very short pulse with very little energy (in the range of nanojoules) and peak power of less than a megawatt. The pulse is then put through a stretcher—a telescope of unity magnification connecting two antiparallel diffraction gratings. The longer the wavelength of light, the shorter the travel path and the sooner pulses arrive at the next stage. The process essentially stretches a pulse as short as 5 fs by up to 105 times to as much as a nanosecond.
The pulse, now with perhaps less than a watt of peak power, is then fed into a medium of high energy density such as Ti:sapphire, which amplifies the pulse significantly—up to the limit of several gigawatts imposed by nonlinearities. This limit, however, is far surpassed when the amplified pulse is recompressed by a pair of parallel gratings. Recompression allows a pulse to reach a length of a few femtoseconds with peak power in the petawatt range (1015 W).
Not only are far-higher powers achieved with chirped pulse amplification, but lasers can be thousands of times more compact for the same energy output, because component materials have energy densities thousands of times greater than that of dye lasers. Bench-top terawatt lasers are thus feasible. In addition, pulse stretching and compression are added before and after the main amplification stage, so the technique can be back-fitted to existing high-power lasers with relatively small expense. Terawatt lasers such as Nova at Lawrence Livermore National Laboratory (Livermore, CA), for example, can be converted to petawatt lasers with subpicosecond pulses.
Compact x-ray sources
Among the many areas of intense research that ultrashort laser pulses have made possible is the effort to develop compact x-ray lasers or other sources of coherent x-ray radiation. Combining the high spatial resolution of x-rays with the high time resolution of ultrashort pulses provides a tool for investigating fast phenomena at the molecular level, including the details of biochemical processes occurring within living cells.
In the last year or so, several teams of researchers have shown that it is possible to generate coherent, fast x-ray pulses by reflecting ultrashort laser pulses from the boundary between a plasma and a vacuum. The production of x-rays occurs through the interaction of visible photons with relativistic electrons in the plasmas. The photons are Doppler-shifted by the electrons, producing a spectrum of higher harmonics with wavelengths down to 20 nm or shorter. The relativistic plasma is produced by the femtosecond pulses at intensities greater than 1018 W/cm2. At the Institute für Laser und Plasmaphysic (Essen, Germany), for example, 35-fs laser pulses focused on a glass target produced up to the 35th harmonic, with the beams of each harmonic diverging no more than the incoming laser beam (see Fig. 1).1While coherent x-rays can reach energies up to 0.5 kV, incoherent bursts of x-rays can extend to much-higher energy. At laser intensities from 2 × 1018 to 1019 W/cm2, electrons can be heated to several hundred kiloelectronvolts, emitting continuum Bremstrahlung radiation as they interact with ions. When researchers at the University of California (San Diego, CA) focused a tabletop laser emitting 28-fs, 80-mJ pulses at a moving copper wire, they measured 300-keV temperatures with a scintillation detector, which allowed them to plot the x-ray spectrum (see Fig. 2).2With the tightly focused spots, effective temperatures approached 1 MeV. In addition, the scientists generated line radiation at 8 keV. Such narrow line radiation, even if incoherent, can be important in x-ray diffraction studies of fast chemical reactions because the source size is essentially the same as that of the laser spot—only a few microns across.
Fast lasers, fast ions
Ultrashort laser pulses can accelerate ions as well as electrons to high temperatures, a process of interest for both thermonuclear-fusion research and efforts to develop compact particle accelerators. Using lasers at the relatively modest intensity of 3 × 1016 W/cm2, a research collaboration between the Institute of Plasma Physics and Laser Microfusion (Warsaw, Poland) and the Institute for Laser Physics (St. Petersburg, Russia) measured the energy of the ions ejected from a copper target. The scientists found that the ions fell into two sharply differentiated groups: a larger one with a velocity of around 740 km/s and a smaller one with a velocity of 2700 km/s, corresponding to maximum energies of 29 and 150 keV, respectively. Such high energies are sufficient to induce fusion reactions in a variety of fuels.
While focusing a pulse on a solid target accelerates ions by thermal processes, in other cases the huge electrical fields generated by ultrashort pulses do the trick. Researchers at the Center for Ultrafast Optical Science at the University of Michigan (Ann Arbor, MI) focused 400-fs, 6-TW lasers to an intensity of 6 × 1018 W/cm2 within a supersonic helium gas jet. The laser pulse self-focused further as it moved through the gas, creating a long narrow channel. This occurred as the laser pulse expelled electrons from the channel, while accelerating them to relativistic velocities and creating an optical lens out of the plasma. As the electrons left the channel, a strong electric field built up and accelerated the ions to move after them. The result was the acceleration of 500-keV ions with high efficiency—nearly 5% of the laser pulse energy converts to fast ion energy.
Light faster than light?
One of the most exotic applications of ultrashort pulses involves the study of superluminal propagation—velocities that seem to be faster than the speed of light in a vacuum (c). While superluminal velocities for matter or energy transfer are prohibited by special relativity, a pulse peak can exceed this velocity in certain circumstances and has been observed doing so in experiments.
Typically, this phenomenon involves tunneling or evanescent waves produced by regions in which the pulse decays exponentially instead of oscillating. According to theory, tunneling speed or evanescent wave propagation should exceed c. Nevertheless, the ability of the pulse peak to exceed c does not mean that energy, and thus information, can travel faster than c and thus violate special relativity.
Ultrashort pulses are needed to study this phenomenon, because the time delays involved are so small—generally femtoseconds. The development of femtosecond terahertz pulses in the far-infrared has enabled a number of such studies, some with surprising results.
Klaas Wynne and colleagues at the University of Strathclyde (Glasgow, Scotland) performed experiments with frustrated total internal reflection in which a single-cycle terahertz pulse is sent through an air gap between two prisms where an evanescent wave is expected (see photo on p. 209).3 Measurements showed that the pulse crossed the gap with essentially zero time delay, moving more than a pulsewidth ahead of a pulse traveling at c. This implied that some energy exceeded the speed of light, thus violating special relativity. However, Wynne believes that further analysis will show that the phenomenon cannot be used to transmit information at faster than the speed of light and therefore is not a true violation. Research in this field is continuing at a rapid pace, and more-sophisticated tests of the predictions of both special relativity and quantum mechanics will be taking place in the near future.
Ultrashort pulses also are finding applications in micromachining, optical spectroscopy, and biomedicine, where the short pulse time minimizes heating of adjacent tissues and allows precision microsurgery. In the next few years, this new tool will likely become useful in laboratories, hospitals, and even factories.
REFERENCES
- A. Tarasevitch et al., Proc. Quantum Electronics and Laser Science Conference '99, 93 (May 1999).
- J. Badziak et al., Lasers and Particle Beams 17, 323 (July 1999).
- K. Wynne and D. A. Jaroszynski, Opt. Lett 24, 23 (June 1999).
About the Author
Eric J. Lerner
Contributing Editor, Laser Focus World
Eric J. Lerner is a contributing editor for Laser Focus World.