Superresolution Fluorescence Microscopy: Finessing photons to improve nanoscopy
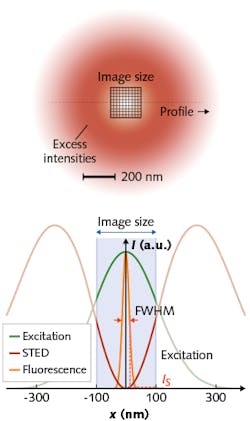
At one of the three Biomedical Optics Symposium (BiOS) plenary sessions during SPIE Photonics West 2018, Stefan Hell of the Max Planck Institute (Gottingen, Germany) discussed concepts enabling new capabilities in far-field optical superresolution microscopy (a.k.a. fluorescence nanoscopy). Hell, of course, was among the recipients of the 2014 Nobel Prize in Chemistry. The new concepts—MINFIELD,1 MINFLUX,2 and DyMIN3—were designed to overcome the barriers that have prohibited superresolution techniques from reaching molecular resolution.
Stimulated emission depletion (STED) fluorescence microscopy, the technique for which Hell is known, enables an observer to distinguish adjacent molecules by sequentially turning their fluorescence off (or on) using a donut-shaped light beam that confines molecular emission to subdiffraction-sized regions. While the discovery of this approach was exciting, it imposed its own limitations since it quickly photobleaches specimens.
With STED, as with other superresolution methods that involve sequential switching of light-activatable probes, the molecules fluoresce (or do not) in regions where light intensity reaches or exceeds a threshold, and they remain in the opposite state in areas where intensity is lower. Triggering a molecule’s switch from fluorescence to nonfluorescence (or vice-versa) requires the intensity pattern maxima to exceed the threshold by a factor that scales with resolution—which, on subdiffraction scales, is large. Achieving the highest resolution means applying high intensities, and creation of an image means exposing each molecule in the specimen to the maxima until it loses its ability to fluoresce. In areas where features are spaced far enough apart that molecules could be distinguished with lower intensity, higher intensity has no purpose.
That’s where the new concepts come in.
Scan smaller
MINFIELD leverages the fact that the high intensities of the donut crest are not necessary for fluorescence switching. True to its name, MINFIELD mitigates loss of fluorescence capability by restricting scanning to subdiffraction-sized regions (see figure). By exposing molecules only to the lower intensities needed for switching, MINFIELD largely avoids transitions to the harmful higher states.
Hell and his team have demonstrated a 100-fold reduction in photobleaching, in fact, which has enabled resolution of details to <25 nm in situations where conventional scanning failed to acquire sufficient signal. And because stronger signal means faster recording times, the researchers predict that MINFIELD-STED will enable a new range of life-sciences applications.
Minimal photon fluxes
While sequential switching makes adjacent molecules discernible, it does not identify their locations in space, which is another necessity for superresolution imaging.
Superresolution imaging techniques fall into two camps in terms of how they identify spatial location. In coordinate-targeted approaches such as STED and reversible saturable/switchable optical fluorescence transitions (RESOLFT), the device controlling placement of the light pattern’s intensity minimum is able to identify the emitter’s position. Approaches such as photoactivated localization microscopy (PALM) and stochastic optical reconstruction microscopy (STORM), on the other hand, estimate emitter position using a camera to detect the centroid of the fluorescence diffraction pattern. While centroid-based methods imply precision to about 5 nm, a variety of factors challenge this target.
MINFLUX is a concept for establishing the coordinates of a molecule with (minimal) emission fluxes.
Like PALM and STORM, MINFLUX relies on stochastic switching of fluorophores, but like STED it locates the emitter using a donut-shaped excitation beam. It probes the emitter using a local intensity minimum of excitation light. Finding the point of minimal emission reduces the number of photons required for nanoscopy: The researchers report needing 22X fewer fluorescence photons (versus centroid localization) to achieve on the order of 1 nm precision and resolve molecules just 6 nm apart.
For single-particle tracking, MINFLUX enabled a 100-fold improvement in temporal resolution and number of localizations per trace. Because they have not reached conceptual limits, the researchers anticipate that the modality will “break new ground for observing the dynamics, distribution, and structure of macromolecules in living cells and beyond.”
Adaptive illumination
Designed to support the coordinate-targeted approaches like STED and RESOLFT, DyMIN (Dynamic Intensity Minimum) generalizes and expands on concepts such as MINFIELD to further maintain the integrity of specimens. It dynamically adapts to apply only as much light as is needed to image specific structural features at the desired resolution.
Assume that you’d like to discern two fluorophores located closer together than the diffraction limit. DyMIN would start scanning in confocal resolution at a distance from the fluorophores. As the flank of the Gaussian excitation spot approaches the dye molecule, though, the detected signal increases. DyMIN boosts the light enough to keep the fluorophore turned “off,” given the enhanced resolution. When the fluorophore emits again at another scan location, illumination increases again, and again until the STED beam matches the target resolution and the two fluorophores appear as separate entities. DyMIN subsequently decreases intensity as it moves to areas less crowded with fluorescent molecules.
By tuning illumination intensity as each pixel/voxel is imaged, DyMIN records fluorescence wherever fluorophores are found within a subresolution “neighborhood,” matching the size of the neighborhood to the structures being imaged. Research demonstrates that DyMIN lowers light dosing to a scanned region up to about 20X under common bioimaging conditions, and more than 100X for sparser 2D and 3D samples. Besides a lowered risk of photobleaching, the benefits include a boost in signal, contrast, and resolution. And bleaching reduction can be converted into accordingly brighter images at better than 30 nm resolution.
REFERENCES
1. F. Göttfert et al., Proc. Nat. Acad. Sci., 114, 2125–2130 (2017).
2. F. Balzarott et al., Science, 355, 606–612 (2017).
3. J. Heine et al., Proc. Nat. Acad. Sci., 114, 37, 9797–9802 (2017).
About the Author
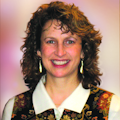
Barbara Gefvert
Editor-in-Chief, BioOptics World (2008-2020)
Barbara G. Gefvert has been a science and technology editor and writer since 1987, and served as editor in chief on multiple publications, including Sensors magazine for nearly a decade.