Photonic Frontiers: Microcavities - The strange world of microcavity optics
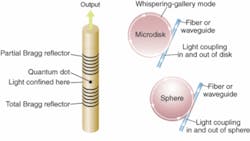
Optical microcavities are micrometer-size resonators that can trap light on a scale comparable with its wavelength, strongly coupling the electromagnetic wave with matter and creating novel effects. In the research laboratory, microcavities are playgrounds to explore topics such as quantum electrodynamics (QED), the manipulation of quantum states by electromagnetic fields. But the novel effects created in microcavities also open the door to new types of lasers and production of highly nonlinear devices such as all-optical switches.
Microcavity research has evolved rapidly over the past decade and the terminology has evolved as well. The new strongly coupling optical microcavities have high Q factors that trap light, which, because of the small cavity size, resonates on a few widely spaced resonances. Quantum dots are no longer considered as microcavities because they are weakly coupling and don’t trap their emission, which leaks out freely as luminescence. However, a microcavity can contain a quantum dot.
In semiconductors, the strong confinement of a microcavity can create a quasiparticle called a polariton, a hybrid of a single photon and an exciton (electron-hole pair) in the solid. Other types of microcavities also exist, but this article focuses mostly on semiconductor microcavities and polaritons.
High-Q microcavity types
Several types of high-Q microcavities have been demonstrated. Their performance depends both on the Q factor and their volume, which often is measured as the cube of the wavelength divided by the refractive index of the cavity material, (λ/n)3. Kerry Vahala of the California Institute of Technology (Pasadena, CA) groups them into three basic families.1
Fabry-Perot microcavities somewhat resemble a VCSEL (vertical-cavity surface-emitting laser) shrunk to micrometer scale. One simple example is a semiconductor micropost or micropillar design in which light oscillates vertically between a pair of Bragg gratings as in a VCSEL (see Fig. 1, left). However the pillar or rod is much thinner, and confines light in a volume that can be as small as several cubic wavelengths.
Whispering-gallery cavities trap light by “continuous total internal reflection” within a high-index ring or sphere surrounded by lower-index material (see Fig. 1, left). Reflection is extremely efficient and Q factors can be extremely high, although the smallest volume cavities usually have lower Q factors. Output coupling is through the edge of the disk or surface of the sphere; often with a waveguide passing close enough to the surface for evanescent-wave coupling.
Photonic crystals are a third alternative, with light able to propagate in the cavity at wavelengths that are blocked by the surrounding material. Output coupling depends on the photonic-crystal geometry.
Some devices do not fit neatly into any single category, such as a microcavity fabricated from a short length of microstructured holey fiber.2
Polaritons and microcavities
Excitation of a semiconductor inside a microcavity generates an electron-hole pair, which recombine as an exciton, emitting a photon that is confined in the microcavity. When the microcavity size matches the emitted wavelength in the solid, the photon is reabsorbed by the same electron-hole pair before it interacts with anything else. The hybrid is a polariton. “At this stage it becomes impossible to discriminate between a cavity photon and an electron-hole pair because energy rapidly oscillates back and forth between them,” says Jeremy Baumberg, now at the Nanophotonics Centre at the University of Cambridge (Cambridge, England).The hybrid nature of polaritons makes them bosons, particles that can share the same quantum state. This leads to properties, including extremely high optical gain and huge nonlinearities, which Baumberg initially measured in gallium arsenide (GaAs) while working at the University of Southampton (Southampton, England). He also used the combination of high gain and high nonlinearities to build a micrometer-scale GaAs optical parametric oscillator.
Polaritons in GaAs dissociate above 50 K, limiting its practical potential, so Baumberg turned to gallium nitride (GaN), which can sustain polaritons at room temperature. Last year, while still at Southampton, his group demonstrated the first room-temperature semiconductor polariton laser using GaN material from the Institute of Quantum Electronics and Photonics at Ecole Polytechnique Federale de Lausanne (Lausanne, Switzerland).3 His polariton laser resembles an optically pumped VCSEL, but for proper coupling the pump light must be incident on the microcavity at a specific angle (see Fig. 2). The polaritons remain in the cavity, but their photon component leaks out through the cavity mirrors, producing a laser beam.
An important feature is that polaritons emit photons from their lowest state, so their population does not have to be inverted to sustain laser action. Baumberg reported that the pump power threshold of 1 mW observed for the first GaN polariton laser corresponded to an absorbed energy density an order of magnitude lower than the best quantum-well VCSELs made from the same nitride materials.
Extending polariton lasers
Optimization of the cavity promises further reductions in pump power, and a thresholdless laser is possible in principle because polariton lasers do not require a population inversion. The trick is to design a cavity in which emission can only be stimulated into one mode, says Rolf Binder of the University of Arizona (Tucson, AZ). “That way, any light you generate is light you generate in the laser mode.”
The basic microcavity design can be optimized in different ways for different purposes. David Press and colleagues at Stanford University (Stanford, CA) last year achieved single-photon emission by resonantly pumping a quantum dot in a strongly coupled micropillar cavity.4
So far, polariton lasers have all been optically pumped. But late last year Jacqueline Bloch’s group at the CNRS Laboratory of Photonics and Nanostructures (Marcoussis, France) demonstrated electrically driven spontaneous polariton emission from a GaAs semiconductor pillar microcavity.5 They call their device a “polariton light-emitting diode,” and say it “opens the way toward new sample designs suitable for the realization of electrically pumped polariton lasers.”
Nonlinearities and strong coupling
The strong coupling of electromagnetic waves and matter produces strong nonlinearities, making polaritons attractive for nonlinear devices. Because the nonlinearity is extremely strong, the devices can be very small. Baumberg says his early optical parametric oscillators were only a few hundred nanometers long, compared to many centimeters for conventional devices.The involvement of matter in polaritons enhances the normally weak interactions between photons, opening up possibilities such as all-optical switching. Binder’s group has proposed using four-wave mixing instabilities that alter polariton scattering in a semiconductor cavity. As in conventional optical phase-matching, the trick is to adjust the angle between the weak switching beam and the strong pump beam to conserve both energy and momentum, so the weaker beam can deflect the more powerful one. Binder’s group has demonstrated the concept in theory, and is seeking an experimental verification.6
Whispering-gallery microcavities
The Q factors of whispering-gallery microcavities can be much higher than those of Fabry-Perot microcavities. Shapes include spheres, toroids, disks, and rings, with spheres having the advantage that surface tension of a liquid droplet can create the extremely smooth surface needed for high Q. Radiation leakage increases with smaller sizes for spheres, but smaller sizes concentrate the circulating light in a smaller volume.
Semiconductor whispering-gallery microcavities can produce polaritons. A team at Fudan University (Shanghai, China) earlier this year reported producing polaritons at room temperature in the tapered arm of a tetrapod made of zinc oxide, a wide-bandgap semiconductor.7 However, most whispering-gallery cavities are made of nonconductors such as silica or quartz and studied for other purposes.
Two interesting demonstrations deserve mention to illustrate some possibilities. One is a photon turnstile, in which a single atom controls propagation of light in opposite directions around a microtoroid, demonstrated by Jeff Kimble’s group at Caltech.8 A second, by Matt Eichenfield of Oskar Painter’s lab at Caltech, showed that the optical dipole force from light coupled between a tapered fiber waveguide and a microdisk can pull the waveguide toward the disk (see Fig. 3).9
Outlook
The future offers many intriguing questions. “Microcavity polaritons provide an exciting opportunity for studying light-matter interactions under unique conditions,” says Leonid Butov of the University of California at San Diego (La Jolla, CA). One current debate is whether polaritons can form a solid-state Bose-Einstein condensate at room temperature. Baumberg thinks prospects are good because polaritons are bosons, but Butov thinks the coherent state of polaritons is closer to a laser than to a condensate. The final results are not yet in.
Most work remains in the laboratory, with material limitations restricting many experiments to cryogenic temperatures. Developers are trying to tame practical issues such as the quality of GaN materials and electrical excitation of polaritons. But the prospects are encouraging. “The consistent progress seen over the last seven years indicates the rich potential in basic science and novel technologies awaiting polariton devices,” says Baumberg.
REFERENCES
1. K.J. Vahala, Nature 424, 839 (Aug. 14, 2003).
2. S.M. Hendrickson, T.B. Pittman, and J.D. Franson, IEEE J. Lightwave Technology 25, 3068, (October 2007).
3. S. Christopoulos et al., Phys. Rev. Lett. 98, 126405 (March 23, 2007).
4. D. Press et al., http://arxiv.org/abs/quant-ph/0609193 (2006).
5. D. Bajoni et al., Phys. Rev. B 77, 113303 (2008) (DOI: 10.1103/PhysRevB.77.113303).
6. S. Schumacher et al., Arxiv.org:0712.2060v.1 (Dec. 12, 2007).
7. L. Sun et al., Direct Observation of Whispering Gallery Mode Polaritons and their Dispersion in a ZnO Tapered Microcavity. Physical Review Letters 100, e156403 (16 April 2008) doi:10.1103/PhysRevLett.100.156403.
8. B. Dayan et al., Science 319, 1062 (Feb. 22, 2008).
9. M. Eichenfield et al., Nature Photonics. 1, 416 (July 2, 2007).
About the Author
Jeff Hecht
Contributing Editor
Jeff Hecht is a regular contributing editor to Laser Focus World and has been covering the laser industry for 35 years. A prolific book author, Jeff's published works include “Understanding Fiber Optics,” “Understanding Lasers,” “The Laser Guidebook,” and “Beam Weapons: The Next Arms Race.” He also has written books on the histories of lasers and fiber optics, including “City of Light: The Story of Fiber Optics,” and “Beam: The Race to Make the Laser.” Find out more at jeffhecht.com.