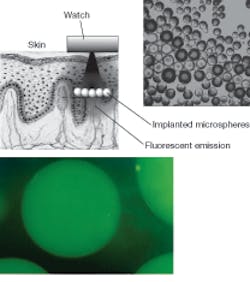
It has been nearly five years since the events of 9/11, and in that time governments worldwide have invested heavily in all things related to homeland security. Among the technologies that have benefited from this trend are optical imaging and optical sensing. While the initial intent was to bolster the ability to detect potentially dangerous air- and water-borne bioagents and pathogens, these same efforts have also enhanced biomedical applications of optical sensors, from glucose monitoring to early cancer detection.
“After 9/11, several special programs were established through agencies such as the National Science Foundation (NSF) and the National Institutes of Health, so if you had biomedical projects, you could adapt them for biodefense,” said Gerard Cote, director of the Optical Biosensing Lab at Texas A&M University (College Station, TX). “This infused the field with more funds and provided a shot in the arm toward sensor technology in general. It also spawned a number of projects designed to bring lab-on-a-chip technologies to the bedside.”
And funding continues to roll in. In April a team of researchers headed by Holger Schmidt at the University of Santa Cruz (California) received a four-year, $1.6 million grant from the National Institute of Biomedical Imaging and Bioengineering to further develop biosensors based on antiresonant reflecting optical waveguides (see www.laserfocusworld.com/articles/218569). And in May, a new sensor research facility dubbed MIRTHE (Mid-infrared Technologies for Health and the Environment) was established at Princeton University (Princeton, NJ) to further the development of optical sensors based on quantum cascade lasers, using $3 million in funding from the NSF (see “MIRTHE center aims to take mid-IR sensors to new heights,” p. 27).
Multiple methods
Optical biosensors are designed to detect the presence of molecules on a surface, in a fluid, or in the air, either by determining changes in light absorption in the sample or measuring the light output via luminescence. A biosensor typically comprises three main components: a sensitive biological element such as a tissue sample or antibody, a transducer, and a detector. Depending on the desired target and the type of sensing technique used, the sensor can take on any number of configurations—from chips to fiberoptic probes—and utilize all kinds of light sources, from white light to diode lasers and LEDs.
Currently, most optical-biosensing applications utilize one of four techniques: near-infrared spectroscopy, Raman spectroscopy, fluorescence spectroscopy, and polarization. Each has certain advantages and disadvantages, especially when it comes to critical factors such as sensitivity and specificity. For example, fluorescence is usually associated with some sort of implantable device, which makes it an invasive approach. However, while the other techniques are noninvasive, they lack the specificity that is possible with fluorescence. Compared to electrochemical, thermal, or pressure-based sensors, optical biosensors have many desirable features, including being extremely sensitive, noninvasive, and nondestructive to the sample.
These days optical biosensors are being developed for a number of patient-based applications, including glucose monitoring, cancer diagnostics, viral and bacterial disease detection, Alzheimer’s detection, and bedside monitoring. Of these, glucose monitoring is the most well-established and commercially successful application. At Texas A&M, the Optical Biosensing Lab is working to develop an in vivo polarimetric glucose sensor with a near-IR broadband light source. Its goal is to noninvasively determine blood glucose concentrations in diabetics by measuring the amount of rotation of a light beam’s polarization state after propagation through the aqueous humor within the anterior chamber of the eye.
Cote’s group—in conjunction with researchers from Penn State and the University of Maryland at Baltimore—is also working with near-IR, Raman, and fluorescence spectroscopy for additional biosensing applications, such as the detection of beta amyloid for diagnosing Alzheimer’s and the detection of analytes in cells. Their ultimate goal is to create chip-level sensors for diagnostics and patient monitoring (see Fig. 1). Current projects include the development of a chip-level sensor that uses surface-enhanced Raman spectroscopy to increase the sensor’s sensitivity. The sensor relies on inelastic scattering combined with a chemical placed near a roughened metal surface or near gold or silver nanospheres, resulting in micromolar (1/1000000 molecular weight per liter), nanomolar, and eventually femtomolar measurements.
“In terms of specificity, spectroscopy is a nice approach because you get multiple peaks that help differentiate the background,” Cote said. “Sensitivity is more complicated; when it comes to Alzheimer’s, for example, you are looking for a protein but you don’t know exactly what concentration you are looking for. Basically you want to know if there is any at all, so you have to use something like surface-enhanced Raman spectroscopy.”Researchers at the Center for Photonics Technology at Virginia Polytechnic Institute and State University (Virginia Tech; Blacksburg, VA) are fine-tuning fiberoptic-based sensing techniques for rapid diagnostics and for detecting bacteria and DNA—eventually down to the cellular level. According to Kristie Cooper, research assistant professor at the center, they are using Fabry Perot interferometers built inside the fibers. The sensor itself is an optical fiber with a thin precursor film that is several layers deep, with each layer attached to the fiber through a layer-by-layer electrostatic self-assembly process. When bacteria, for example, are present, their antigens are attracted to the antibodies, causing the organism to attach to the fiber. When exposed to a white light that travels through the fiber, this process causes a change in the light that escapes through the fiber wall (see Fig. 2). The resulting interference spectrum is then measured using refractometry transduction.
“We don’t use labels-we are just looking at the binding of the molecule or bacteria itself,” Cooper said. “Instead of using a single cavity on the end of the fiber we use multiple cavities so we can detect changes in a very thin cavity. We put a thin film on the end of each cavity that contains a receptor. As it combines with the target, you see an increase in the thickness of the cavity. The size of the thickness change helps us determine the concentration.”
While the probe is currently able to glean only “yes/no” results, Cooper and her colleagues say the complexity of the information obtained from the sample could be increased by multiplexing several fibers together to look at multiple organisms at the same time, with each fiber corresponding to each strain of bacteria or DNA sequence.
Next-generation sensors
Other groups are adapting even more-sophisticated biosensing technologies to increase sensitivity and create chip-level sensors that can move many of these applications out of the laboratory. For example, Claire Gmachl, associate professor of electrical engineering at Princeton and director of the MIRTHE center, and colleagues are focusing on quantum-cascade lasers for chemical sensing in the mid-IR range. Potential biomedical applications include breath analysis for the detection and monitoring of kidney and liver disease and oxidative stress.
“Chemical vapors have very strong absorption features in the mid-IR range,” Gmachl said. “So if you have optical techniques in the 3 to 30 µm range (roughly), then you can do optical sensing of chemicals. And the quantum-cascade laser uses a semiconductor material that is very mature, which gives the whole sensing endeavor a reliable foundation. In addition, laser foundries already exist that can produce them in volume.”
At UCSC, a desire to optically detect molecules one at one time led Schmidt and his colleagues-in collaboration with Aaron Hawkins at Brigham Young University (Provo, UT)-to develop micron-scale single-mode integrated optical waveguides for biosensing applications ranging from virus detection and ribosome observation to molecular biology and flow cytometry (see www.laserfocusworld.com/articles/218569). In 2004 they reported the first demonstration of integrated optical waveguides with liquid cores. This technology, using the principle of antiresonant reflecting optical waveguides, enables light propagation through tiny volumes of liquids on a chip. Since then they have optimized the waveguides for use in sensor devices by attaching fluidic reservoirs that enable the user to control the movement of the molecules. Next they plan to combine the waveguides with nanopore devices to create a new sensor that can provide both electrical and optical characterization of single biomolecules, with all the components ultimately integrated onto a single chip.
“Our goal was to combine integrated optics and microfluidics, where there are all these channels and you can make highly functionalized systems on a chip,” Schmidt said. “By adding the integrated optics you can guide the light through the liquid channels on the chip, so everything is planar and you don’t need a microscope.”On the commercial front, one company is convinced that surface plasmonics offers a key advantage for biosensing-notably the ability to create tiny spectroscopic sensors that aren’t just portable, but are mobile and even wearable (see www.laserfocusworld.com/articles/229675). NanoLambda (Pittsburgh, PA), a spinoff of the University of Pittsburgh, is developing the Spectrum Sensor, built on metallic “nano-islands” such as a nanowire array, in which the scale of the wire and the wire-to-wire gap is in nanoscale (see Fig. 3). The s ensor chip size is a few millimeters on a side. According to the company, each pixel of the chip detects a predefined wavelength of light from an input source, yielding a spectral fingerprint for each material being imaged. While initial applications are expected to be chemical sensing and high-resolution RGB (red/green/blue) color sensing, NanoLambda says the sensor will also be used for blood diagnostics, biochips and wearable health monitors.
“Because of wavelength-specific plasmonic-resonance phenomena between lights and metals, the metallic nanowires can work as a wavelength filter. We can design these structures for each wavelength, fabricate an array for multiple wavelengths together, then monolithically integrated onto a detector array, it becomes a high-resolution spectrometer on a chip,” said Bill Choi, CEO of NanoLambda. “As we learned from the experience of MEMS industry, small size itself is not enough for commercialization. It should be mass-producible at low cost. Since we are using standard wafer processes to fabricate the metallic nanostructures, the cost of Spectrum Sensor will be very affordable even for the consumer mobile devices. Our goal is to make the optical spectroscopic capability ubiquitous, make available wherever you need it.”
Imaging our world
The best way to have control of our circumstances is to be aware of them. Optical sensing can measure (and image) objects and scenes around us to exquisite precision; it can also detect and quantify the various substances in our surroundings that are both beneficial and deleterious to us.
In the first article of this Optoelectronic World supplement, contributing editor Kathy Kincade outlines several leading-edge developments in biomedical spectroscopic and fiberoptic sensors, some of which descend from military devices. James Seipmann of LightTime then describes a compact real-time 3-D ladar system that combines advantages of two existing approaches. Finally, Tim Day and his colleagues at Daylight Solutions highlight the application of a broadly tunable IR quantum-cascade laser to chemical sensing.
John Wallace
Senior Editor
About the Author
Kathy Kincade
Contributing Editor
Kathy Kincade is the founding editor of BioOptics World and a veteran reporter on optical technologies for biomedicine. She also served as the editor-in-chief of DrBicuspid.com, a web portal for dental professionals.