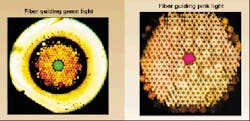
The idea of the photonic bandgap is opening new possibilities in optical fiber design. Light-guiding in conventional optical fibers depends on total internal reflection at the boundary between a high-index core and a lower-index cladding. Fiber properties can be refined by changing core and cladding materials, by adjusting their interface, and by adding extra layers, but the range of possibilities remains limited. Many new possibilities arise when internal microstructures are incorporated into the glass fiber, creating new types of fibers variously known as holey or photonic crystal fibers. Photonic bandgap fibers are an important subcategory.
Microstructured fibers can have properties quite different from those of conventional fibers. One family of holey fibers guides light through hollow cores, something impossible in conventional fibers, which require a high-index core and low-index cladding to guide light by total internal reflection (see photo at right). This approach offers the potential for very low attenuation and chromatic dispersion. Alternative designs offer other special properties. Some transmit light in a single mode across a range of wavelengths and core diameters much larger than otherwise possible. Other designs concentrate light in a very small effective area, increasing nonlinear effects greatly for use in new types of optical devices. The technology is young, and new possibilities continue to emerge.Photonic bandgaps
The key idea behind many of these new fibers is the photonic bandgap, a region that blocks the passage of light at certain wavelengths. Photonic bandgaps are analogous to the electronic bandgaps of semiconductor materials, which block the flow of electrons that lack a minimum energy. Electronic bandgaps arise from the gap in electronic energy states between the valence bands (occupied by electrons that form atomic bonds) and the higher-energy conduction band (occupied by electrons that carry current through the semiconductor).
Photonic bandgaps arise from the interaction of lightwaves with microstructures in an optical material. One simple example is a stack of alternating layers of two transparent materials with different refractive indexes. Reflection from the interfaces between the layers scatters certain wavelengths out of the stack, effectively blocking them from the material. In this way, a photonic bandgap can guide or confine light by restricting it to certain layers. Figure 1 shows a two-dimensional photonic bandgap material, in which three sets of layers at different angles overlap to block light from entering the structure at a range of angles of incidence.
A true photonic bandgap fiber uses this elaborate structure to confine light radially and guide it along the length of the fiber. It consists of a cylinder of photonic bandgap material like that in Figure 1, surrounding a central hole or other region through which light can travel. The mutually intersecting layers produce a pattern that looks just like an array of regularly spaced holes, and in practice a set of parallel holes running the length of the fiber creates a two-dimensional photonic bandgap. The properties of the material depend on the size and spacing of the holes.
As in conventional fibers, light-guiding requires two types of material: the photonic bandgap material serves as the cladding, and a material with different structure is needed for the core. One possibility is a void or empty region. Another possibility is a region with different microstructure, either a different pattern of holes or a solid region. All are called photonic crystal fibers, but those with solid cores do not rely strictly on the photonic bandgap effect to guide light.
Holey fibers are produced using techniques adapted from older technology for drawing bundles of fused fibers for imaging. Bundle production proceeds in a series of stages. First glass rods are stacked and fused together, then drawn into composite rods called multifibers. Then the multifibers are stacked together and the process is repeated, producing a solid block of glass fiber, with all the interstices squeezed out.
Production of holey fibers starts instead by stacking hollow tubes, then drawing them into a fused bundle that preserves the internal holes. Glass rods also may be used, with interstices intentionally left among them. The fibers are drawn together to produce a fine composite rod, which may be stacked with similar rods and drawn again to make a thin fiber. Instead of squeezing out any interstitial air, the process leaves holes open. In practice, drawing reduces the fiber diameter around 10,000 times, and can leave holes as fine as 25 nm running the length of the final fiber.
Many variations have been developed, but the two primary types are fibers with hollow and solid cores.Hollow-core fibers
Conventional fibers cannot guide light through a hollow core—the optical equivalent of a metal microwave waveguide—because total internal reflection requires that the core index be larger than the cladding. Photonic bandgap materials can confine light in a hollow core, however.
Figure 2 shows light guiding in hollow-core photonic bandgap fibers. With the proper structure, reflections from the layers surrounding the core combine to produce constructive interference when light is directed inward, concentrating light in the hollow core. In contrast, light leaking outward is attenuated. The result is to guide light through the fiber's hollow core, an effect possible only with photonic bandgaps to confine the light in the low-index zone. These are true photonic bandgap fibers.
Light transmission depends on resonance effects within the structure, which confine certain wavelengths but not others. Experiments at the University of Bath (Bath, England) showed a transmission spectrum with a series of peaks of low attenuation separated by regions where attenuation is much higher.1 Another effect of the structure is that the hollow core transmits only a single transverse mode, although its diameter is much larger than that of a normal single-mode fiber core.
The hollow-core fibers are made by drawing stacks of hollow tubes down into thin fibers, with the central tubes removed from the bundle. Experiments have shown that removing one central tube is not enough. A core group of seven must be removed in order to support a single mode, as predicted by theory. Removing more cores allows multimode transmission.
Hollow-core photonic bandgap fibers look quite attractive for telecommunications. Guiding light through air rather than glass promises very low levels of dispersion and nonlinear effects. Hollow-core fibers also may offer lower levels of attenuation. Versions with small cores could be used to concentrate light in order to enhance laser interactions with materials trapped inside the core for applications such as harmonic generation and Raman amplification.
Coaxial waveguide fibers
Photonic bandgaps also can be adapted to make the optical equivalent of a coaxial cable. The idea grew from experiments at the Massachusetts Institute of Technology (MIT; Cambridge) that showed that multilayer photonic bandgap structures could serve as omnidirectional mirrors, without the angular sensitivity of conventional thin-film mirrors. Developers then envisioned rolling the layer to form a cylinder, and running a rod of photonic bandgap material down the center.
Theoretical analysis showed that this structure could guide light like a coaxial cable guides radio waves.2 Developers point out that the coaxial structure offers two important advantages over conventional fibers. One is a lack of polarization sensitivity, because the fundamental mode of the coaxial "omniguide" is radially symmetric, and consistent along its length. The second is the ability to guide light around much sharper turns than a conventional fiber. So far such fibers have not been demonstrated.Solid-core holey fibers
Other photonic crystal fibers have solid cores. They fall into two general categories: types with true photonic bandgap guidance, and types functionally similar to conventional fibers based on total internal reflection. One way to visualize the difference is by considering the effective refractive index of the material to be an average over the solid glass and the holes it contains. The larger the volume of holes, the lower the effective refractive index will be. Thus if a holey fiber has a solid core, the effective refractive index of the holey cladding is lower than that of the solid core, and the fiber is a modified total-internal-reflection type. If there are more holes in the core, its effective refractive index is less, and light-guiding depends on the photonic bandgap.
The structural difference is quite evident in Figure 3. The hollow-core photonic bandgap fiber (top) was drawn from an array of hollow tubes with a hexagonal hole left in the center by seven missing tubes. It has the advantage of guiding light through air, where dispersion and nonlinear effects are low. In the total-internal-reflection structure (bottom), the core is a solid block surrounded by holey material that has a lower effective refractive index, and the index difference confines light in the core. Both structures guide light in glass, but their internal structures can be engineered to give them properties not available in conventional fibers. Wide variations are possible.
Solid-core holey fibers transmit light in a single mode through much larger cores than are possible in conventional fiber, because single-mode operation depends on geometry alone. In one early experiment, researchers guided 458-nm blue light through a core 22-µm across—an effective area some 20 times larger than possible in conventional single-mode fibers.3 In these fibers, the holes are relatively small, and most of the cross-section is glass. Fibers with larger air holes can provide very high dispersion, for use in dispersion compensation and management.
Alternatively, a solid core can be suspended at the center of a grid-like core that looks like a spider web in cross-section. This approximates the properties of a thin glass fiber suspended in air. Suitable designs can shift the zero-dispersion point over a wide range of wavelengths, an important capability for dispersion management.4
Other patterns of holes can shrink the effective area of a holey fiber to as little as 1 µm2 at 1550 nm, according to simulations performed at the University of Southampton (Southampton, England). Fibers have been made with effective areas as small as 2.8 µm2 at 1550 nm.5 These small effective areas concentrate power, greatly enhancing the strength of nonlinear effects in glass, so nonlinear devices based on holey fibers could be 10 to 100 times shorter than similar devices using conventional fiber.
Prospects for a young field
Like other young technologies, holey fibers are full of both promise and uncertainty. Important new results continue to emerge from both theorists and laboratories."Photonic crystal fibers have opened the door to numerous new applications within the areas of fiberoptics and waveguiding," says Anders Bjarklev of the Technical University of Denmark (Lyngby), who gave an invited paper at OFC '01 in March (Anaheim, CA). He warns that the technology "is still very young, and we have only seen a few experimental demonstrations of fibers." Yet he also cites the promise of "completely new properties, such as spectrally wide operation, improved spotsize control, [and] wavelength selectivity."
ACKNOWLEDGMENTS
Thanks to Anders Bjarklev, Philip St. John Russell, and Periklis Petropoulos for comments and advance copies of papers. I would also like to thank Philip St. John Russell for his help with the art.
REFERENCES
- R. F. Cregan et al., Science 285, 1537 (Sept. 3, 1999).
- M. Ibanescu et al., Science 289, 415 (July 21, 2000).
- J. C. Knight et al., Elec. Lett. 34, 1347 (1998).
- J. C. Knight et al., IEEE Phot. Tech. Lett. 12, 807 (2000).
- P. Petropoulos et al., OFC 2001, paper TuC3.
About the Author
Jeff Hecht
Contributing Editor
Jeff Hecht is a regular contributing editor to Laser Focus World and has been covering the laser industry for 35 years. A prolific book author, Jeff's published works include “Understanding Fiber Optics,” “Understanding Lasers,” “The Laser Guidebook,” and “Beam Weapons: The Next Arms Race.” He also has written books on the histories of lasers and fiber optics, including “City of Light: The Story of Fiber Optics,” and “Beam: The Race to Make the Laser.” Find out more at jeffhecht.com.