Tunable Sources: Choosing a tunable ultrafast laser system
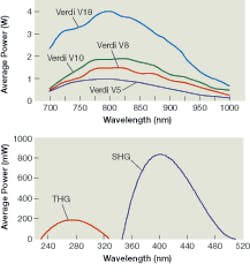
Applications of ultrafast (femtosecond and picosecond) laser systems often require the ability to tune or shift the output wavelength. Laser manufacturers have responded to this need with a wide range of interrelated products that reflect the diverse range of applications for tunable pulses-together with various options and accessories, the entire product family tree can appear quite complex. A comparison of the relative merits of three basic system architectures that deliver tunable output can simplify the process of choosing a particular system configuration to meet an application need.
Ti:sapphire tunable oscillators
In a modelocked laser system, the shortest possible pulsewidth is determined by the Fourier transform of the spectral bandwidth of the pulse; a wider spectral bandwidth supports shorter pulses. Titanium-doped sapphire (Ti:sapphire) offers the widest gain bandwidth of any currently available material, enabling systems to deliver pulsewidths shorter than 10 fs and making it the material of choice for today’s state-of-the-art ultrafast laser systems.
The broad gain bandwidth of Ti:sapphire also provides another important advantage for many users: the ability to tune the center wavelength of the output pulses over this bandwidth (see Fig. 1). The simplest tunable ultrafast system is thus a Ti:sapphire oscillator pumped by a 532 nm solid-state continuous-wave (CW) laser. Moreover, the same basic Ti:sapphire laser can be configured for either femtosecond or picosecond operation by constraining the spectral bandwidth.
In dispersive materials such as lenses, Ti:sapphire crystals, and even dielectric coatings, the different wavelength components of an ultrafast pulse travel at slightly different velocities. The effect of this group velocity dispersion (GVD) is to stretch the overall temporal profile of the laser pulse, becoming a critical concern in the femtosecond range. These effects of GVD must be compensated to achieve the shortest possible (transform limited) output pulse. A simple way to do this is to incorporate a matched pair of dispersive prisms within the Ti:sapphire laser cavity. The prisms serve to make the effective cavity length a function of wavelength and can be used to create a GVD effect that is identical in magnitude, but opposite in sign, relative to the cumulative effect of the other optical elements in the laser cavity.
The Ti:sapphire laser is tuned by rotating a birefringent filter that acts as a bandpass filter for the polarized intracavity beam. If the center wavelength is changed by a few tens of nanometers or less, that is the only adjustment required. For large changes in wavelength, one of the prisms in the prism pair must be adjusted because cavity GVD changes with wavelength.
The pulse repetition rate is very high for a modelocked Ti:sapphire oscillator, with a typical value of 76 MHz. Depending on the pump power used, the output power of a Ti:sapphire oscillator can exceed 4 W, which translates into pulse energies at the 50 nJ level. But because of the short pulse duration, the peak power can be as high as 0.5 MW. This enables harmonic conversion and other nonlinear wavelength-shifting techniques to be used to extend wavelength coverage-important because the typical Ti:sapphire oscillator has a spectral gain bandwidth covering only 680 to 1090 nm. Utilizing the second harmonic accesses the 350 to 540 nm wavelength range and the third harmonic delivers 225 to 330 nm. Furthermore, the pulse energies of second-harmonic and third-harmonic generation can be significantly enhanced by cavity dumping the Ti:sapphire oscillator. This “bolt on” accessory for the Ti:sapphire oscillator boosts the oscillator pulse energy by up to 10 times, albeit with a corresponding reduction in pulse repetition rate.
Ti:sapphire oscillators support a range of applications depending upon system wavelength and power. The low pulse energy is well suited to condensed-phase experiments in physics, chemistry, biology, and electrical engineering. The high sample density means that the signal can exceed the shot noise even with nanojoule pulses, and the extremely high repetition rate allows statistical averaging over many millions of pulses to be achieved in only seconds. Conversely, “tunable” applications that can need more than just a Ti:sapphire oscillator include gas-phase experiments in which the signal would be less than the laser shot noise, and/or experiments requiring wavelengths in the infrared or in the so-called “Ti:sapphire gap” between 500 and 700 nm.
Synchronously pumped OPOs
The simplest way to access the Ti:sapphire gap and/or wavelengths longer than 1 µm is to use a synchronously pumped optical parametric oscillator (OPO) in conjunction with the Ti:sapphire oscillator. An OPO relies on a nonlinear process called parametric down-conversion, which can be considered to be the opposite of sum-frequency mixing, and thus requires a nonlinear crystal. In an OPO, an input “pump” photon is split to produce two photons of lower energy, referred to as the signal and idler photons, in which the sum of the two photons conserves the original photon energy. By convention, the high-photon-energy (shorter-wavelength) output is referred to as the signal beam; the idler is the lower-energy photon beam. The OPO process also conserves photon momentum, which requires a phase-matching condition and determines the wavelength of the signal (and hence idler). The phase-matching condition can be adjusted by changing the pump-laser wavelength, the angle of the nonlinear crystal, or the crystal temperature-the three methods by which the output(s) of an OPO is tuned.
Like a Ti:sapphire laser, the OPO can be operated in the femtosecond or picosecond domain. But unlike a laser, the OPO crystal does not store any optical gain-it will only emit light during the time it is pumped. For this reason, the cavity of an ultrafast OPO is set to the same length as the Ti:sapphire cavity, or for the same 76 MHz repetition rate. Every time the signal pulse circulating in the OPO passes through the nonlinear crystal, this crystal is simultaneously being pumped by a pulse from the Ti:sapphire oscillator, delivering gain to the signal and idler beams within the OPO cavity (hence the name “synchronously pumped OPO”). During each pass around the OPO, part of the signal beam leaves the cavity as an output beam through a partially reflecting cavity optic.
There are many variants on the basic OPO format, two of which merit further examination. The first is the use of an optional ring-cavity configuration. This approach generates a high-power intracavity signal beam. An intracavity doubler is then used to convert this near-infrared beam to a visible wavelength, delivering high power over the entire Ti:sapphire gap.
The second variation utilizes periodically poled OPO crystals, which have two advantages compared to bulk crystals such as potassium titanyl phosphate (KTP). Most important, the OPO can be automatically tuned by adjusting the OPO output coupler to lengthen (or shorten) the cavity (see Fig. 2). In femtosecond operation, for example, the pump wavelength is fixed at approximately 830 nm for OPO tuning over a 500 nm infrared range. In contrast, bulk-crystal OPOs require tuning of the Ti:sapphire oscillator wavelength. In addition, the periodically poled material provides direct access to wavelengths as long as 3.3 µm. Even longer wavelengths can be generated by difference-mixing of the signal and idler beams.As with the basic Ti:sapphire tunable oscillator, the subnanojoule pulse energy of a tunable OPO means these sources are best suited to applications involving condensed-phase samples. A common application involves the fast-growing field of carbon nanostructures (tubes, spheres). Here the absorption bandgap is a function of the precise nanodimensions, so each structure has its own optimum interrogation wavelength.
Amplifiers and tunable OPAs
For low-signal ultrafast experiments such as gas-phase photophysics and photochemistry, much higher pulse energies are generally required to raise signal levels over shot noise. This need is typically met by kilohertz-repetition-rate ultrafast amplifiers in conjunction with OPAs (optical parametric amplifiers). These systems are also preferred for mid-IR experiments at wavelengths up to 20 µm.
Ultrafast amplifiers with kilohertz pulse repetition rates can be based on either regenerative or multipass amplification. In both instances a Pockels cell injects a single pulse from a Ti:sapphire oscillator into the amplifier which contains a Ti:sapphire crystal pumped by a powerful pulsed green neodymium:yttrium lithium fluoride (Nd:YLF) laser. These amplifiers routinely produce ultrafast pulses at millijoule power levels and custom amplifiers can reach up to ten millijoules. These pulses can then be used to drive one or more tunable OPAs.
An OPA makes use of the same nonlinear optical process as an OPO, but because the pump pulses have higher peak power, an optical resonator is not required to achieve efficient conversion. As with an OPO, the wavelength of the output is again controlled by the phase-matching conditions of the crystal, which can be tuned by changing the angle of the crystal. With this arrangement, a nominal 1 mJ input can be converted into a tunable 100 µJ pulse-more than sufficient pulse energy for complex nonlinear mixing and harmonic schemes that provide continuous wavelength coverage anywhere from 200 nm to 20 µm (see Fig. 3). Even at these extremes of the tuning range, this type of arrangement can deliver microjoule pulse energies.To the uninitiated, these appear to be very complex systems: a Ti:sapphire modelocked laser and its 532 nm pump laser, a Ti:sapphire amplifier and its pulsed pump laser, followed by one or more OPAs. Modular systems like this have tremendous flexibility, but for end users just needing tunable output at one or more wavelengths, laser manufacturers such as Coherent (Santa Clara, CA) also offer “one-box” solutions in which all the lasers needed to generate the amplified ultrafast pulses are integrated in a single turnkey box. Because the same software suite that operates this box also controls one or more OPAs, a single computer automatically optimizes every aspect of system operation, from the pump-diode temperatures to the wavelengths of multiple OPAs and even their harmonics modules.