Photonic Frontiers: Light-emitting Diodes: LEDs are workhorses with applications far beyond lighting
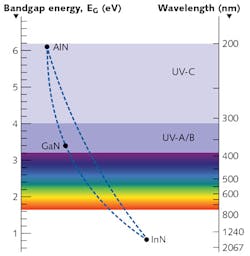
Light-emitting diodes (LEDs) have come a long way from the arrays of red dots that served as numbers on the first pocket calculators. Today's glaring spotlight is on LED lighting, but LEDs are exploring a broad range of other frontiers. Advances in nitride semiconductors have pushed commercial LEDs well into the 200–280 nm ultraviolet-C (UV-C) band. Near-UV LEDs can deliver watt-scale output for applications ranging from sensing and adhesive curing to phototherapy. On the research frontier, micron-scale LEDs are powering investigations in optogenetics, and developers are exploring prospects for germanium-tin (GeSn) LEDs in silicon photonics.
The UV LED boom
Nitride semiconductors have spawned a boom in UV as well as blue light sources. The bandgap of pure gallium nitride (GaN) corresponds to 365 nm in the UV. Adding indium increases the wavelength to produce violet and blue LEDs. Adding aluminum decreases the wavelength, pushing LEDs deeper into the UV. As shown in Fig. 1, pure aluminum nitride (AlN) has a bandgap wavelength of 210 nm, which NTT Basic Research Laboratories (Atsugi-shi, Japan) reached a decade ago, and which remains the shortest LED wavelength.1 Since then, the big improvements have been in power and performance.
UV LED power and efficiency are highest at 360–400 nm, where the active layer is largely GaN. These devices typically are grown on sapphire (Al2O3) substrates, although GaN and silicon carbide also are used. Maximum outputs of commercial single-emitter LEDs are in the watt range.
Power and efficiency drop sharply at wavelengths shorter than about 350 nm. Some LEDs are available in the UV-B band from 280 to 315 nm, but there is more interest in the UV-C band at 200–280 nm, which has stronger bioeffects and is more attractive for important types of sensing. DARPA's Compact Mid-UltraViolet Technology (CMUVT) program, completed two years ago, sought better LEDs in this range for applications including sensing and water purification. Milliwatt-class commercial LEDs now are available from 240 to 280 nm.
The choice of substrate is a crucial for deep-UV performance. As the aluminum concentration increases, reducing the LED wavelength, the lattice mismatch with sapphire grows larger. Aluminum nitride is harder to grow, but provides better lattice matching for UV-C LEDs, says Hari Venugopalan, director of global product management at Crystal IS (Green Island, NY). He says AlN substrates are a key factor in the company's ability to offer LEDs with output above 10 mW at 250–280 nm. They are now sampling 230 nm LEDs, which will be a commercial first.
UV-C LED applications
The new generation of UV-C LEDs is attractive for environmental quality measurements long performed by deuterium, xenon, or mercury discharge lamps. LEDs have longer lifetimes and lower replacement costs. They don't yet meet the power requirements for disinfection in drinking-water plants, which largely have shifted from chlorine chemical treatment to UV treatment with intense 254 nm mercury lamps. But LEDs are well suited to monitoring absorption in the 255 nm band to ensure proper irradiation levels.
LEDs also can monitor treatment of wastewater, a problem in India where 40% of the treated water does not meet national standards. Ensuring compliance requires a network of UV-C-based sensors to measure and report BOD and UV absorption levels every 15 minutes. UV-C LEDs can meet those requirements more reliably and less expensively than deuterium lamps or chemical measurements systems, says Venugopalan.
High nitrate levels become a major issue for U.S. water systems because they can cause algal blooms that generate toxins and contaminate drinking water. This has led the Alliance for Coastal Technologies, the Environmental Protection Agency, and other organizations to sponsor a challenge program seeking new nitrate and phosphate sensors that can collect real-time data unattended for at least three months, and cost less than $5000. Nitrates absorb strongly at 230 nm, says Venugopalan, "so LEDs would be ideal candidates."
UV-C LEDs also can protect optical and acoustic sensors used in coastal or marine environments from fouling by biofilms that grow on hard surfaces. As little as 2–3 mW of light at 260–275 nm can deactivate DNA in the bacteria that that form the layers, keeping surfaces clean. As available powers increase, UV-C LEDs could be used for larger-scale treatments such as water purification or germicidal treatment.
LEDs emitting at 280 nm can measure absorption by uric acid to monitor the progress of kidney dialysis in real time, which previously required time-consuming blood tests. Another use of 280 nm LEDs is exciting fluorescence in oil that has leaked into water. The emitted wavelength can identify the type of oil, which is important for determining what dispersants to use to clean up the spill.
Near-UV LED applications
More powerful LEDs emitting in the near-UV are replacing lamps for curing adhesives and inks. LEDs last over 40,000 hrs. compared to 1000–8000 hrs. for mercury lamps and consume much less electricity, lowering operating costs.
Typically, adhesives are cured with 365 nm LEDs to take advantage of photoinitiators originally developed for mercury lamps. The deep penetration of that wavelength eases full-depth curing. The ability of LEDs to focus light onto small areas is a big advantage for electronics manufacturing because it can avoid stray light damaging sensitive components, increasing production yields of products such as touch-screen panels, says Mark Gaston of Excelitas Technologies (Waltham, MA).
Printers prefer 395 nm for ink curing because they have developed special inks to take advantage of the higher LED powers available at that wavelength, says Mike Kay of Excelitas. The high powers available from 365 to 395 nm make LEDs at those wavelengths attractive for exciting fluorescence spectroscopy in a wide range of materials.
Phototherapy
Near-UV LEDs are promising for destroying pathogens in blood used for transfusion, says Gaston. Now used mainly in Europe, the process is based on adding a compound called amotosalen to the blood and activating it with a near-UV source.2 This kills pathogens, but does not damage blood plasma and platelets as long as they are not illuminated by shorter UV wavelengths. Shifting from the fluorescent lamps now used to 350 nm LEDs would kill the pathogens, control the process better, and reduce heating of the blood, he says.
Phototherapy also extends into the visible, with blue LEDs now being used to treat jaundice in infants by breaking down a yellowish compound called bilirubin, which can accumulate to dangerous concentrations without treatment. Babies had been exposed to fluorescent lamps. Blue LEDs are more efficient, can be matched to the 458 nm peak absorption of bilirubin, and also can be connected to fiber-optic blankets, so the baby can be wrapped in the "biliblanket" and illuminated by blue light delivered through the fibers (see Fig. 2).Micro-LEDs on the research frontier
Micro-scale LEDs are playing important roles on research frontiers such as optogenetics, the use of light to control neurons or other cells in living tissue. The cells are genetically modified to express light-sensitive "opsin" proteins, and to allow monitoring of their response. The technique allows probing neural activity in living animals, and was listed as one of the breakthroughs of the decade in Science.3
Delivering light precisely to individual neurons has been a challenge. Fiber-optic probes are large compared with neurons, and can limit movement of mice used in experiments. Planar waveguide probes required fiber tethering or diode laser sources. Now, György Buzsáki of the New York University Neuroscience Center (New York, NY) and Euisik Yoon of the University of Michigan (Ann Arbor, MI) have shown that a micro-LEDs can deliver a broader range of wavelengths and couple more directly to neurons (see Fig. 3).4Germanium-tin LEDs
Another emerging technology is compound GeSn LEDs fabricated on silicon. Their allure is that blending about 10% tin into germanium can produce a semiconductor with a direct bandgap, offering far more efficient light emission than possible in other indirect-bandgap Group IV materials. Output is around 2 μm.
The field is young and demonstrations are hard. So far, a few groups have made LEDs and the only lasers made were optically pumped and operated well below room temperature. But in a review paper, Erich Kasper and Michael Oehme of the University of Stuttgart (Stuttgart, Germany) concluded that further work should lead to development of efficient LEDs.5
REFERENCES
1. Y. Taniyasu, M. Kasu, and T. Makimoto, Nature, 440, 325–328 (2006).
2. J. Irsch and L. Lin, Transfus. Med. Hemother., 38, 1, 19–31 (Feb. 2011); doi:10.1159/000323937.
3. Science news staff, "Stepping away from the trees for a look at the forest," Science, 330, 6011, 1612–1613 (2010).
4. F. Wu et al., Neuron, 88, 1136–1148 (Dec. 16, 2015); http://dx.doi.org/10.1016/j.neuron.2015.10.032.
5. E. Kasper and M. Oehme, Jpn. J. Appl. Phys., 54, 04DG11 (2015); http://dx.doi.org/10.7567/JJAP.54.04DG11.
About the Author
Jeff Hecht
Contributing Editor
Jeff Hecht is a regular contributing editor to Laser Focus World and has been covering the laser industry for 35 years. A prolific book author, Jeff's published works include “Understanding Fiber Optics,” “Understanding Lasers,” “The Laser Guidebook,” and “Beam Weapons: The Next Arms Race.” He also has written books on the histories of lasers and fiber optics, including “City of Light: The Story of Fiber Optics,” and “Beam: The Race to Make the Laser.” Find out more at jeffhecht.com.