OPTOELECTRONIC APPLICATIONS: BIOPHOTONICS - Fiber lasers find opportunities in medical applications
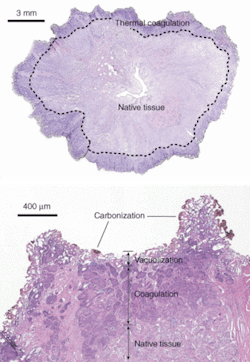
Fiber lasers have become one of the hottest topics in photonics in recent years, particularly as the technology has grown in robustness and reliability. But most of what we read or hear about these lasers is related to industrial and telecom applications involving powers in the 1- to 2‑kW range. Some research groups and commercial entities, however, are pursuing lower-power fiber-laser applications in medicine, such as optical coherence tomography (OCT), microsurgery, and skin resurfacing. In fact, they anticipate that fiber lasers will increasingly replace more-established light sources in medical applications, thanks to their lower cost, inherent user-friendliness, broad range of energy delivery (pulsed and continuous wave), and applicability for both surgery and diagnostic imaging.
“For surgical applications it is important that the laser be compact, low maintenance, and efficient,” said Stuart Jackson of the Optical Fibre Technology Center (OFTC) at the University of Sydney (Australia). “The high efficiency and good thermal management of fiber lasers make them very suitable for surgical applications, and because they are diode-pumped, they are also compact. The fiber geometry has an additional advantage over bulk solid-state lasers because it alleviates the need for an additional delivery fiber, thus reducing cost and system complexity.”
Invented in 1963 by Elias Snitzer, the fiber laser required almost two decades of development before it finally appeared in the commercial world (see “Subwavelength optics come into focus,” p. 86, and Laser Focus World, August 2002, p. 83). Early iterations used single-mode diode pumping and emitted only tens of milliwatts but still attracted users because of their large gains and the feasibility of single-mode CW lasing for many transitions of rare-earth ions not achievable in crystal lasers. The jump to watt-level fiber-laser output occurred in 1990 when a 4-W erbium-doped fiber laser was reported.1 This development laid the groundwork for the 10-W and higher single-mode fiber lasers we see today.
In fiber lasers, the optical fiber itself acts as the resonator cavity. These devices typically comprise a single-mode fiber core doped with erbium, ytterbium (or a combination thereof), or thulium. Energy from a solid-state source is coupled into the fiber’s cladding, then moves into the core and pumps the dopant. In the medical field, the most desirable wavelengths are in the 1.3-µm range for imaging and the 1.5-µm (a water absorption peak) to 4-µm range for surgical applications, at powers ranging from milliwatts to more than 100 W.
“Any biomedical application that uses a CW laser, particularly a high-power CW laser, can benefit from a fiber laser,” Jackson said. “The higher efficiency, wider tunability, and better beam quality over the entire power range offered by fiber lasers are significant advantages; as a result, fiber lasers widen the utility of lasers in general for medical applications.”
Soft-tissue surgery
Recent progress in the development of high-power thulium-fiber lasers, perhaps the most convenient source of high-power 2-µm laser radiation, means that new medical applications for these lasers will emerge, Jackson adds. His group at the OFTC is developing high-power fiber lasers for emission in the 2- to 4-µm region and exploring a number of fiber lasers with the intention of producing more than 20 W at 3 µm, for surgical applications in otolaryngology, urology, ophthalmology, and cardiology.
IPG Photonics (Oxford, MA), which manufactures commercial diode, ytterbium (Yb)-, erbium (Er)-, thulium (Tm)-, and Raman-fiber lasers, started working with clinicians in urology, dermatology, and ophthalmology to apply various types of these fiber lasers for soft-tissue surgery, starting with a 100‑W 1.94-µm thulium-fiber laser that is showing potential for the treatment of benign prostatic hyperplasia and a 1.5-µm erbium-fiber laser for dermatology. Biomedical versions of IPG’s thulium-fiber lasers modules are available in powers up to 100 W at central emission wavelengths selectable from 1.8 to 2.1 µm; these multimode diode-pumped systems can operate in CW and pulsed modes. Earlier this year Nathanial Fried, assistant professor of urology at The Johns Hopkins School of Medicine (Baltimore, MD), reported first feasibility results with IPG’s 110-W Tm-fiber laser operating at 1.9 µm, rapidly vaporizing prostate tissue at a rate of 0.83 ± 0.11 g/min and with a thermal coagulation zone measuring 500 to 2000 µm and thus demonstrating the potential for hemostasis (see Fig. 1).2
“This research found that the 110-W thulium-fiber laser is capable of rapid soft-tissue incision, vaporization, and coagulation,” he said. “The ability of the thulium-fiber laser to operate at or near the 1.94-µm water-absorption peak in tissue may also provide improved precision in cutting tissues compared to the holmium and KTP lasers.”
According to Fried, although further studies are needed, “the high-power thulium-fiber laser is a promising new laser technology that may find its niche in medicine as a single, compact laser platform capable of performing multiple applications, including precise incision, rapid vaporization, and adequate coagulation for hemostasis during soft-tissue procedures.” Other potential advantages include smaller size, more efficient operation, improved spatial beam quality, and, as a result, potentially more precise tissue incision.
Researchers at the University of Manchester (England) are also working with thulium-fiber lasers for medical applications, such as pain stimulation. In collaboration with some hospital medical groups, Terry King and the university’s Photon Physics Group have developed a 2-µm Tm-silica-fiber laser tunable from 1.8 to 2.05 µm for laser stimulation of cutaneous nerve fibers in conjunction with functional imaging (EEG, PET, and fMRI) for human pain research. King and his colleagues are also working with other CW and pulsed fiber lasers in the 1- to 3-µm range, including Yb-silica, Yb-Er-silica, Er,Pr-ZBLAN (praseodymium-ZBLAN), and a coupled Yb,Er-silica and Tm-silica system for optical coherence tomography (OCT).
Erbium-fiber lasers
In fact, several academic and commercial groups are working with erbium-fiber lasers for OCT and surgical applications. According to these researchers, high-performance, short-coherence-length light sources with broad bandwidths and high output powers are critical for high-speed, ultrahigh-resolution OCT imaging. For example, James Fujimoto at the Massachusetts Institute of Technology (Cambridge) and Wolfgang Drexler at Medical University (Vienna, Austria), pioneers in the OCT field, are currently working with a fiber-laser prototype designed by Roy Taylor’s group at Imperial College (London, England) specifically for OCT. According to Taylor, the OCT sources—which are based on a CW ytterbium fiber laser, operate around 1.3 µm, and generate up to 500‑mW output power at bandwidths ranging from 400 to 700 nm—offer several advantages over the short-pulse femtosecond lasers typically used in OCT, including being more compact (A4 footprint), robust, and affordable, with no need for optical alignment, while still achieving axial resolution of less than 5 µm (see Fig. 2).“Most people like to operate around 1.3 µm because you have less scatter there and also good penetration before it hits the water-absorption peak,” Taylor said. “We shift the wavelength through nonlinearly using a Raman process. But the big advantages here are that it is all continuous-wave Yb-fiber-laser pumped, relatively high power, and an extremely broadband source, which means you get very high resolution. So you can shape the spectrum and you can afford to throw power away because the power is almost free, particularly with the CW fiber lasers.”
Higher-power erbium-fiber lasers in the 2- to 3-µm range also show promise for microsurgery applications. Spire (Bedford, MA) has demonstrated a 1.2‑W, 2.7-µm CW erbium-doped fluoride glass-fiber laser, optically pumped by a 980-nm laser diode and is now embarking on a second National Institutes of Health-funded study of fiber lasers in glaucoma surgery (see Fig. 3). Other areas of active research include vitreoretinal surgery, myringotomies (a small incision made in the eardrum to treat ear infections), and other types of microsurgery. According to the company, the advantages of using the fiber laser in microsurgery include smoother cuts and faster incision and excisement.“This laser has much potential for microsurgical applications in areas where you need a very small and moderately low-power laser,” said Kurt Linden, senior scientists at Spire Biomedical. “In vitreoretinal surgery, researchers have used the Nd:YAG laser to remove blood clots and blood-infested tissue close to the retina, but this approach leaves spots in the tissue because it is not possible to make continuous cuts. In myringotomies, physicians have used the CO2 laser, but it makes a very loud ‘pop’ (from the shock wave) when it fires, which can scare the patient. With the fiber laser, you can ramp it up and avoid the shock wave.”
Erbium fiber lasers are also at the heart of the most established commercial fiber laser in the medical arena: the Fraxel SR aesthetic-laser system from Reliant Technologies (Palo Alto, CA). This system, which has U.S. Food and Drug Administration clearance for soft-tissue coagulation, periorbital pigment reduction, sun spots, and skin resurfacing, utilizes a 30-W erbium-fiber laser (from IPG) operating at 1550 nm that works in conjunction with Reliant’s proprietary scanning system to achieve very precise resurfacing and remodeling of the skin while minimizing the collateral thermal damage (see Fig. 4).According to Len DeBenedictis, founder and chief technology officer of Reliant, instead of “bulk treating” the skin, the collimated beam of the single transverse-mode fiber laser enables the scanner to form thousands of very small spots on the treatment surface in a very short period of time. The system is designed to treat about 20% of the skin in a single session; the goal is to treat the face in 20 minutes by creating 1 million “microthermal zones,” each one-tenth of a millimeter across but penetrating hundreds of microns deep into the tissue. The epidermis is then shed and new epidermis created; in addition, the extremely high beam quality of the laser denatures the dermis in a needle-like projection that does not kill the surface tissue. The result, DeBenedictis says, is “nonablative resurfacing.”
“We are the first large-quantity user of fiber lasers in medicine because the medical field hasn’t caught on to this laser yet,” he said. “A lot of other (aesthetic) companies don’t do scanning and don’t make microscopic spots, so they aren’t interested in the high-end fiber lasers. In fact, much of the light used in medical aesthetics is flashlamps that irradiate a large area, so what does it matter if you can focus down to 20 or 30 µm?”
REFERENCES
1. V. Gapontsev et al., Adv. Solid State Lasers Topical Meeting, Tech. Digest, 258, Salt Lake City (1990).
2. N. Fried, K. Murray, The J. Endourology19(1) 25 (2005) and Lasers in Surgery and Medicine36(1) 52 (2005).
About the Author
Kathy Kincade
Contributing Editor
Kathy Kincade is the founding editor of BioOptics World and a veteran reporter on optical technologies for biomedicine. She also served as the editor-in-chief of DrBicuspid.com, a web portal for dental professionals.