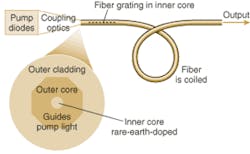
Fiber lasers have become the hotbed of solid-state laser development. The most publicized advances are in high-power fiber lasers, in which the power in a diffraction-limited beam has jumped severalfold in just two years. But the technology is also advancing in other directions, including better ways to generate ultrashort pulses at high powers, and production of wavelengths that had previously been hard to produce by other means.
The driving force is a critical mass of new technology and ideas. Fiber lasers benefit tremendously from steady improvements in pump diodes and the properties of ytterbium-doped fibers. They also benefit from improvements in fiber design and fabrication, new specialty fibers, and other optical technology developed during the telecommunications bubble.
Building blocks
The main building blocks of a rare-earth doped fiber laser are shown in Fig. 1. The fiber itself consists of three layers: an inner core doped with a rare-earth element (usually ytterbium or erbium), an outer core (or inner cladding) of slightly lower refractive index that collects and guides the pump light, and an outer cladding of polymer or glass that confines the pump light within the outer core. A single-mode inner core is preferred for its beam quality. The outer core often is not circularly symmetric. The cavity optics are fiber Bragg gratings fabricated in the inner core.
The dual-core fiber design has become standard because the large outer core can efficiently collect pump light, while the smaller inner core concentrates laser power in a smaller volume for a higher-quality output beam. The geometry of the outer core is chosen for efficient pumping of the inner core. Similar structures are used in pulsed and continuous-wave lasers. In practice, the fiber is coiled in one or more loops. The design details depend on the application and power requirements.
High-power fiber lasers
Some modifications to the basic design are needed for high-power operation. The outer core typically is expanded to collect more pump energy. The inner core also is expanded to improve energy transfer from the outer core and to avoid nonlinear effects and optical damage that can occur at very high power densities. However, too large a core produces multimode emission, which reduces beam quality. This has led to development of large-mode-area fibers, which support a single transverse mode but have a core diameter larger than those of standard single-mode fibers.
The maximum core diameter D that can maintain single-mode transmission at a wavelength λ depends on the core and cladding index, and is given by:In standard single-mode fibers the index difference is about 0.5%, corresponding to a numerical aperture (NA) of about 0.1, and the core diameter is around 9 µm. The maximum core diameter for single-mode transmission can be increased by reducing the refractive-index difference, but this also reduces light-guiding in the core, increasing bend losses. Numerical aperture can be decreased to about 0.06 before bend losses become unacceptable.
Near-single-mode operation is possible in fibers with somewhat larger cores if they are coiled so higher-order modes experience much higher losses than the fundamental mode. The effect works well with fibers with 20-µm inner cores and 400-µm outer cores, which are widely used in high-power fiber lasers although they are not strictly single-mode if straight. Using coiled 20-µm core ytterbium-doped fibers, Almantas Galvanauskas of the University of Michigan recently reported generating 700 W in a single transverse mode.1 It may be possible to use 30-µm cores with suitable optics.
Further scaling up of output power requires coherently combining the outputs of multiple fiber lasers, which must be linearly polarized. The most straightforward approach is to make double-clad doped fibers that contain internal strain, which causes them to maintain a single linear polarization. Strain-inducing members are formed in the outer core, where they sit on opposite sides of the inner core.Photonic-crystal fiber lasers
Larger single-mode core diameters are possible in photonic-crystal fibers, where light guidance differs fundamentally from standard fibers. The core in this case is a solid region surrounded by a microstructured cladding, formed by drawing a fiber from one or more solid rods surrounded by hollow tubes. The holey region has a lower effective index than the solid core it surrounds, so the structure forms a single-mode waveguide. Good confinement has been demonstrated with cores as large as 30 µm.
This technique has been extended to making fiber lasers by placing solid rods doped with ytterbium at the center of the stack. Jens Limpert and colleagues at Friedrich Schiller University in Jena, Germany, have produced 80-W emission with a photonic-crystal fiber laser with mode-field diameter as large as 21 µm.2 An “air cladding” of large holes surrounded the photonic-crystal outer core, which was diode pumped (see Fig. 2, top). It’s also possible to make polarization-maintaining microstructured fibers by adding larger holes near the core, (see Fig. 2, bottom).3
Researchers at the University of Sydney recently added an unusual twist by making a microstructured polymer fiber version of a solid-state dye laser.4 Alexander Argyros and colleagues drilled a pattern of holes into a preform of commercial PMMA (polymethyl methacrylate), drew it into an intermediate preform, then filled the holes with a solution containing Rhodamine 6G. The solvent partly dissolved the PMMA matrix, so the dye molecules permeated it before it was drawn into the final fiber. Their design left an 18-µm core surrounded by a low-density holey region that they operated as a pulsed laser.
Femtosecond fiber lasers
Femtosecond laser developers have also turned to fiber lasers as they try to improve the technology for generating ultrashort pulses. Control over fiber dispersion properties is invaluable in chirped-pulse amplification, in which pulses are dispersed in time, amplified, then compressed in time to produce very high peak powers. Fiber oscillators can be made very compact, then plugged into a series of amplifiers to boost output power to high levels while maintaining short pulse length. Ytterbium has the broad gain bandwidth needed to generate short modelocked pulses, and its wavelength is midway between those of titanium-sapphire and erbium-fiber lasers. A broad range of ultrafast fiber lasers is under development to meet the diverse needs of the field.
One goal is to produce femtosecond pulses at a high repetition rate for applications in biological imaging, micromachining, and telecommunications. The natural modelocking repetition rate is inversely proportional to the cavity length, so it is limited by the lengths of fiber used. Harmonic modelocking can multiply the repetition rate by producing multiple pulses that circulate within the laser cavity. Active modelocking produces picosecond pulses, so passive modelocking is needed to reach the femtosecond regime. In experiments at the University of Rochester, Wayne Knox and Yujun Deng have used a semiconductor saturable absorber in a colliding-pulse configuration with a ytterbium-doped fiber laser. The saturable absorber is placed at 1/14 of the laser cavity length from a resonator mirror to produce modelocked 380-fs pulses at 605 MHz, the 14th harmonic of the laser’s fundamental modelocking frequency, while suppressing modelocking at other frequencies.5
Active harmonic modelocking of picosecond pulses has been demonstrated at 40 GHz, for potential telecommunications applications. For example, a team at National Chiao Tung University in Taiwan used loss modulation of a Fabry-Perot diode laser at 1 GHz to produce 3-ps pulses at a 40-GHz repetition rate from an erbium-doped fiber ring laser.6
Another interesting use of modelocked fiber lasers is generation of multiwavelength “combs” offset from each other by the repetition rate of the laser. An important refinement first demonstrated with Ti:sapphire lasers is phase-locking and control of the base frequency from which the other wavelengths are offset. The technique recently has been extended to erbium-fiber lasers by generating modelocked pulses in a ring oscillator, amplifying them externally, then spectrally broadening them in a highly nonlinear dispersion-flattened fiber to create an octave-wide range of frequencies. Beating the 1100-nm short-wavelength end of the band against the 2200-nm long-wavelength end produces the base frequency.7 Frequency combs are sought for precision measurement, but other schemes can generate multiwavelength emission for telecommunications.
Raman fiber lasers
Classic doped-fiber lasers are limited to operating in the bands in which the dopants have net gain. Raman fiber lasers give much more flexibility, because they oscillate at a wavelength offset from the pump band by the Raman shift, a frequency that depends on the glass host.In the typical arrangement, pump light from a cladding-pumped ytterbium-doped fiber laser emitting near 1100 nm is coupled into the core of a single-mode fiber optimized to generate stimulated Raman scattering (see Fig. 3). Fiber Bragg gratings fabricated in the core serve as the mirrors >for the Raman resonator cavity. Cavities resonant at different wavelengths can be stacked around each other to perform a series of Raman-shifting steps. In this design, the innermost cavity is resonant at the first step from the pump wavelength, so stimulated Raman scattering at λ1 from the ytterbium-pump laser oscillates within it. This first-step Raman-shifted light can, in turn, produce stimulated Raman scattering at a second, longer wavelength, λ2, which oscillates in a cavity formed by fiber Bragg gratings that reflect that wavelength on either end of the innermost cavity. That process can be quite efficient, so additional steps can be added to shift the wavelength to the desired range, determined by the outermost resonator.
The resonator design determines the wavelengths chosen. If the inner cavities confine all the light at the resonant wavelength, and output coupling is only from the outermost cavity, the Raman fiber laser emits at a single wavelength. Multiwavelength output is possible if some of the inner cavities have their own output coupling mirrors as well, so some of the intermediate Raman wavelengths emerge in the output. Tunable fiber Bragg gratings allow adjustment of the output power. Other recent advances include wavelength conversion by four-wave-mixing in a highly nonlinear dispersion-shifted fiber Raman laser.8
Outlook
The frontiers of fiber lasers are expanding rapidly in other directions as well. A wide variety of optical configurations have been demonstrated, including tunable and Q-switched versions. Some configurations have been demonstrated with active materials other than erbium and ytterbium, such as a single-frequency thulium-doped distributed-feedback fiber laser and a single-mode 2.5-W holmium-doped fluoride fiber laser emitting at 2.86 µm.9, 10
The next challenge will be sorting through the many configurations demonstrated in the laboratory to find the best prospects for extending the fiber-laser technology to commercial products.
ACKNOWLEDGMENT
Thanks to Wayne Knox, University of Rochester, for help with femtosecond fiber lasers.
REFERENCES
- A. Galvanauskas, Optics & Photonics News 42 (July 2004)
- J. Limpert et al, Optics Express 1, 818 (April 7, 2003).
- F. C. McNeillie et al, Optics Express 12, 3981 (Aug. 23, 2004)
- A. Argyros et al, Optics Letters 29, 1882 (Aug. 15, 2004).
- Y. Deng et al, Optics Express 12, 3872 (Aug.9, 2004).
- G-R Lin et al, IEEE Photonics Tech. Lett. 16, 1810 (August 2004).
- B. R. Washburn et al, Optics Letters 29, 250 (Feb 1, 2004).
- T. Okuno et al, OFC 2004, paper MF21.
- S. Agger et al, Optics Letters 29, 1503 (Jul 1, 2004).
- S. D. Jackson, Optics Letters 29, 334 (Feb 15, 2004).
About the Author
Jeff Hecht
Contributing Editor
Jeff Hecht is a regular contributing editor to Laser Focus World and has been covering the laser industry for 35 years. A prolific book author, Jeff's published works include “Understanding Fiber Optics,” “Understanding Lasers,” “The Laser Guidebook,” and “Beam Weapons: The Next Arms Race.” He also has written books on the histories of lasers and fiber optics, including “City of Light: The Story of Fiber Optics,” and “Beam: The Race to Make the Laser.” Find out more at jeffhecht.com.