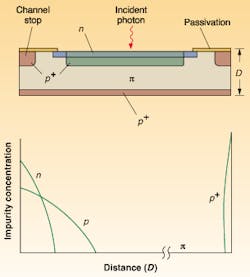
For years, photomultiplier tubes (PMTs) were the main tools used for low-light-level measurement, but times are changing. For many applications, other techniques offer significant advantages. In the infrared range, for example, solid-state avalanche photodiodes offer superior counting capabilities, while superconducting tunnel junctions and microcalorimeters not only count photons, but determine each one's energy as it is recorded, a big plus for astronomical uses. At the same time, though, PMTs are becoming increasingly more sensitive as developers attempt to keep up with the competition.
Avalanche photodiodes
The solid-state equivalent of a photomultiplier tube, which multiplies electrons in a vacuum chamber, is the avalanche photodiode (APD). Here, as in any photodiode, a p-n junction of two semiconductor types allows current to flow in only one direction.
Photodiodes consist of a silicon-doped layer with atoms carrying one valence electron less than silicon (p-type semiconductor) on top of a layer doped with atoms carrying extra valence electrons (n-type semiconductor). Charge migration creates a depletion region with an electric field directed toward the p-region, allowing current to flow in only one direction. With reverse bias potential applied across the diode, the absence of light leads leaves just a dark current produced by thermal generation of electrons. With exposure to light, electron-hole pairs are created, generating a current.
In an ordinary photodiode, the process ends there. In an APD, the bias potential is higher, and the electrons are sufficiently accelerated to ionize the semiconductor atoms, creating new free electrons. The acceleration process then repeats, producing more electron-ion pairs in an avalanche process very similar to that in a PMT.
With the bias potential set just below the breakdown voltage, the APD has a fixed gain. As more and more electrons are accelerated in the avalanche, a space charge builds up, reducing the effective accelerating potential. At some point, electrons, on average, lose more energy to friction than they gain by acceleration and do not reach the high energies required for ionization. The avalanche then dies out, after having given rise to hundreds or thousands of electrons for each initial photon. The current produced by many such avalanches combines into a steady stream measurable with external circuits.
With the bias potential set above the breakdown voltage, the potential remains high enough to overcome the space charge, and the avalanche continues indefinitely, turning into a steady flow of current produced by a single photon. In this mode, the devices are termed single-photon avalanche diodes. Here, the current continues until it is quenched by a decrease in the bias voltage below the breakdown level.
Avalanche photodiodes span a wide spectral range, depending on their primary semiconductor material. Silicon devices are suitable for a range of 400 to 1100 nm, germanium for 800 to 1550 nm, and indium gallium arsenide for 900 to 1700 nm. In areas of the spectrum where the different materials overlap, indium gallium arsenide provides the lowest noise and best frequency response, but the highest price, while germanium is the intermediate option in both respects.
Types of avalanche
While all APDs function basically the same way, three different structures are commercially available: deep-diffusion, reach-through, and the newest, a super-low ionization-ratio type. The deep-diffusion device has the simplest structure, with the n-region resistivity set to produce a very high breakdown voltage (typically around 2 kV), which produces a wide depletion layer. Beveling prevents breakdown at the edges, which reduces field strength there. Because the n-layer is much deeper than the p-layer, electrons produced in the n-layer multiply more readily than holes. This in turn reduces dark current noise, which is mostly generated by holes. The disadvantage is that only light absorbed in the p-layer leads to effective multiplication, so that is the region with the lowest field. As a result, charge accumulates slowly, and response time is greater than 10 to 30 ns.
Overall, the deep-diffusion structure produces slow response and high gain at wavelengths shorter than 900 nm, and fast response and low gain at longer wavelengths. In addition, since some photons are absorbed in the wide n-layer, and are thus multiplied by less than the maximum amounts, there is a fairly large random-shot noise contribution due to varying levels of multiplication.
In contrast, the reach-through structure has a very narrow junction, with multiplication taking place at the back end of the device, farthest from the light source (see Fig. 1). Most of the thickness of the device consists of low-doped silicon or germanium, in which the field strength is very low. Here the photons that have been absorbed take just picoseconds to drift toward the acceleration junction. Since almost no photons are absorbed in the multiplication region, variations in gain are nearly eliminated. All electrons, once they have "reached through" to the p-n junction, undergo exactly the same acceleration and therefore multiplication. While the resulting device offers high-speed response and low noise, it also is more expensive to process.
The third type resembles the reach-through structure, but has a low ionization ratio (the ratio of the number of holes to the number of electrons produced by a given amount of radiation). The low ratio is obtained by gradually increasing the accelerating electric field to a maximum near the deepest point of the device, where practically no hole current exists. An effective k of 0.002 results in a lower noise content. Tests at NASA Langley Research Center (Langley, VA) indicate that these devices are superior to reach-through designs for wavelengths shorter than 950 nm.1
Superconducting tunnel junctions
Another low-light-level detector is the superconducting tunnel junction. Developed in 1996, this device works along entirely different principles than the detector types just discussed. It also offers very high performance. Another advantage comes from the capability to detect both the number of photons absorbed and the energy of each individual photon.
In astronomy, in particular, it has long been a goal of scientists to gather spectral, imaging, and timing information simultaneously, but this has been difficult to do using charge-coupled-device technology. Timing information can only be achieved by short exposures, which cuts sensitivity, while spectral information requires either gratings to spread out the light for a given point source or filters to gain some spectral information over an entire image. Superconducting tunnel junctions, on the other hand, can detect the time of arrival of individual photons, and the number of electrons released by each is proportional to the photon energy. For this reason, an array of the devices can provide all four dimensions of information at once. There is one large catch, though: the junctions, which are limited to operation at very low temperatures (below 1 K), use conventional, instead of high-temperature, superconductors.
A superconducting tunnel junction consists of a sandwich of aluminum oxide between two layers of niobium, or, in some cases, tantalum, with additional layers of aluminum between each niobium or tantalum layer and the central aluminum oxide (see Fig. 2). When the top niobium layer absorbs a photon of radiation, the photon breaks up the Cooper pairs of electrons that maintain superconduction, producing free electrons. The higher the photon energy is, the more free electrons are produced. These free electrons then tunnel across the aluminum-oxide barrier, driven by a bias voltage. A small magnetic field prevents the superconducting Cooper pairs from also tunneling. The electrons trapped in the aluminum layers thus create a current proportional to each photon's energy.As x-ray detectors, superconducting tunnel junctions can provide resolutions as fine as 26 eV for photons in the range of 6 keV. For optical and ultraviolet (UV) detectors, energy resolution is coarser, with photon energies divided into four or five bins between 350 and 650 nm.2 It is possible to better that resolution by at least fivefold, though, and quantum efficiencies can approach 50% across the band from 200 nm to 1 µm.
An alternative and closely related approach involves microcalorimetry, in which a superconductor—held at the transition temperature at which it becomes superconducting—is used as a highly sensitive thermometer.3 If a photon is absorbed by the transition edge sensor, its temperature will increase, greatly boosting its resistance. In general, a feedback loop holds the resistance and thus the temperature constant at the edge of the superconducting range, and the amount of current supplied to a heater then becomes a measure of the energy of the incoming photon. These microcalorimeters can offer energy resolution of 0.2 eV for UV photons in a 4-eV range, with response times of a few microseconds.
In the future, it may be possible to use arrays of such superconducting tunnel junctions on space instruments to produce spectra of thousands of the faint galaxies. Such spectra could yield both the galaxies' distances and the age of their stars, vital data in understanding the evolution of the cosmos. According to Francesco Parco of the European Southern Observatory, "This promises a true revolution in the way astronomers work."
Improving PMTs
Photomultiplier tubes have not been completely knocked out of the single-photon counting game by these newer competitors. In fact, researchers continually seek new ways to improve PMT sensitivity. One such effort at Optische und Elecktronishce System GmbH (Egloffstein, Germany) involves combining an image intensifier with a position-sensitive, crossed-wire PMT to produce a photon-address digital detector system.4
In essence, this type of system is a two-stage PMT (see Fig. 3). In the first stage, the image intensifier (a microchannel plate) multiplies photoelectrons produced by the incoming photon. The electrons then excite phosphors on the rear plate of the image intensifier. These photons in turn are focused onto the position-sensitive PMT. In this way, it becomes possible to enlarge the critical portion of the image, overcoming the relatively coarse spatial resolution of the PMT. Response times again are in the range of microseconds.Since all of these technologies are still rapidly evolving, it is not yet clear which will dominate for rapid photon counting. With technology advancing as fast as it is, though, scientists will soon be able to squeeze almost every bit of information out of even the lowest-level radiation sources.
REFERENCES
- T. F. Rafeat, et al., Opt. Eng. 39, 2642 (October 2000).
- C. M. Wilson et al., Nucl. Inst. And Meth. In Phys. Res. A444, 449 (July 2000).
- P. Verhoeve, Nucl. Inst. And Meth. In Phys. Res. A444, 435 (July 2000).
- M. Pruksch and F. Fleischmannn, Appl. Opt. 39, 3443 (July 2000).
About the Author
Eric J. Lerner
Contributing Editor, Laser Focus World
Eric J. Lerner is a contributing editor for Laser Focus World.