CCD cameras provide a better view for proteomics
The completion of the Human Genome Project marks a fresh starting point for several new research paths. Because genes code for proteins, it is no surprise that proteomics is at the top of the list of emerging disciplines. Scientific and commercial communities have been quick to recognize the tremendous potential that this field of exploration holds for drug discovery, pharmaceuticals, and nanotechnology.
Among the most commonly used investigative components of proteomics are two-dimensional gel electrophoresis, single-molecule fluorescence (SMF), and x-ray crystallography. These three application areas enable researchers to separate and identify proteins, gain insight into protein functionality, and determine protein structure. Although quite different from one another, each relies heavily upon the use of performance-optimized CCD camera systems to provide quantitative data.
Two-dimensional gel electrophoresis
While the human genome comprises 25,000 to 30,000 genes, the proteome is thought to be three to five times larger, because of the many protein isoforms that result from alternate splicing of RNA and from post-translational modifications (phosphorylation, for example). Two-dimensional gel electrophoresis, a method that allows proteins to be separated and identified by isoelectric point and relative molecular weight, is playing a key role in the effort to catalogue this vast set of compounds.
In recent years, high-performance CCD cameras have supplanted film as the imaging tool of choice for the majority of electrophoresis experiments. Intelligently designed digital imaging systems, particularly those incorporating cooled, interline-transfer CCDs, deliver the desired combination of low-light sensitivity, quantum efficiency, dynamic range, spatial resolution, and duty cycle. In addition, these quantitative CCD camera systems can acquire linear, digital data that can be viewed, analyzed, and archived instantly.
There are several indications that the utility of high-performance CCD cameras for two-dimensional gel electrophoresis will continue to increase as the discipline of proteomics matures. First, as implied by the sheer multitude of proteins, highly parallel analysis—in other words, high-throughput imaging—is becoming a more critical requirement. Second, electrophoresis results are often visualized by staining the proteins with a fluorescent dye (or by using a chemiluminescent tag); therefore, good quantum efficiency at the emission wavelength and excellent low-light sensitivity are crucial to these experiments.
Another trend is that more attention is being paid to low-abundance proteins—less frequently expressed proteins whose detection using two-dimensional gel electrophoresis can depend on the sensitivity, dynamic range, and resolution offered by the imaging system. And finally, the ability of high-throughput, low-light CCD cameras to detect and discriminate between multiple-wavelength signals is invaluable to researchers using gel electrophoresis to compare fluorophore-labeled cell populations (see Fig. 1).
Single-molecule fluorescence
Single-molecule fluorescence, which encompasses several specific microscopy techniques, is currently used to investigate various aspects of protein structure and function at the molecular level, such as single-molecule DNA sequencing, the biophysics of protein molecules, and the screening of single-molecule protein microarrays.
One of the more intriguing applications of single-molecule fluorescence is its role in the pursuit of what has been dubbed the thousand-dollar genome. This term describes the goal of one day being able to quickly and completely identify an individual's genome for less than $1000, an endeavor with great implications for the future of personalized health care and pharmacogenomics. From an imaging standpoint, nonamplified DNA targets (base-pair nucleotides) emitting weak fluorescence at four separate wavelengths render this real-time identification process challenging. To address these requirements, high quantum efficiency at each of the emission wavelengths, low-light sensitivity, and fast frame rates are needed.
Another growing area of proteomics focuses on the biophysics of single molecules. Much of fundamental biology is dictated by protein-protein interactions (in essence, the activation and deactivation of proteins). And although proteins tend to act in aggregate, scrutiny at the molecular level reveals details obscured by larger-sample studies. Fluorescence resonance energy transfer (FRET), a dual-label technique that indicates whether two molecules are collocated, is often used to examine these dynamic protein interactions, which include conformational and mobility changes, trafficking and chaperone behaviors, and folding. A high-QE camera system that provides low-light sensitivity, high-speed operation, and good spatial resolution is necessary to collect useful quantitative data.
Protein microarrays, meanwhile, are being used to screen for the presence of low-abundance proteins in small-volume samples from cells. State-of-the-art equipment now enables thousands of biomolecular interactions to be probed in parallel. Of central importance to this application is a high-throughput imaging system with good spatial resolution as well as sensitivity optimized for low-light-level signal detection and quantitation.
The lower fluorophore concentrations and faster kinetics associated with all of these SMF applications establish key criteria for choosing an appropriate digital camera system. Fortunately, on-chip multiplication gain in CCDs now offers researchers a new solution for single-molecule fluorescence.
Basically, on-chip multiplication gain is a signal-boosting technology that provides the sensitivity of intensified CCD and electron-bombardment CCD camera systems, but without the drawbacks (that is, lower resolution, higher cost, and susceptibility to damage from bright light) of external image-intensifier hardware. Instead of using an intensifier, secondary electrons are generated in an extended serial register of the CCD via an impact-ionization process that is initiated and sustained when higher-than-typical clock voltages are applied, effectively reducing the CCD read noise by the on-chip gain factor, which can eclipse 1000×.
The net result is that photon-generated charge is multiplied above the read noise even at supravideo frame rates, a capability that allows the special detectors to meet the requirements of SMF proteomics. To compound the sensitivity advantage provided by on-chip multiplication gain, some of these new CCDs now implement back-illuminated chip architectures that offer better than 90% quantum efficiency. This technology tandem delivers the best available low-light-level sensitivity at fast frame rates and full spatial resolution (see Fig. 2).X-ray crystallography
X-ray crystallography can be used to construct highly detailed structural maps of proteins. For this technique to yield viable data, it is imperative that perfect protein crystals be used. A perfect crystal is one in which individual molecules each have the same shape and orientation, thus allowing incident x-rays to be diffracted in uniform patterns by the molecules' atoms. A series of these diffraction patterns must be acquired from several different angles to obtain sufficient information about the protein crystals.
Although laboratory x-ray sources are sometimes used to study either small molecules or simple protein crystals, both of which require relatively low x-ray intensity to produce a clearly detectable diffraction pattern, these instruments are more commonly used to evaluate the suitability of protein crystals for synchrotron experiments. Unlike laboratory sources, in which nontunable output is characteristic of the element (like copper) making up the anode of the x-ray tubes, synchrotrons are tunable sources that are capable of providing purer monochrome light and much higher beam brilliance, or flux. The ability to deliver a high-flux x-ray beam at any one of a broad range of individual wavelengths or energy levels makes synchrotrons ideal for studying protein crystals of all sizes.
To detect the x-rays from diffraction patterns (see Fig. 3), CCD camera systems use a down-converter, typically a phosphor coating that has been applied to a fiberoptic taper bonded to the CCD. The x-rays strike the phosphor, are converted to a visible wavelength of light (usually green), and conveyed via the fiberoptic taper to the photosensitive pixel array of the CCD.Regardless of the size of a crystal and its molecules, the angular separation of spots occurring in an x-ray diffraction pattern is optimized when 1-Å (12.4-keV) x-rays are used. Taking this fact into account, along with the x-ray camera system's resolution as it relates to the phosphor-coated surface on the fiberoptic taper, the ideal size of the detection plane would be approximately 300 mm in diameter.
Because the largest fiberoptic tapers available measure less than 200 mm in diameter, a significant limitation must be overcome. There are two popular approaches to solving this problem. First, a large taper can be bonded to a large-format CCD (see Fig. 4). Subsequently, during the experiment, the crystal is rotated in such a way that the acquired images can be tiled to show a full diffraction pattern. Alternatively, four (or even nine) large tapers can be bonded to individual large-format CCDs and then bundled together to create a mosaic camera system that affords a 300-mm field of view.The ultimate aim of proteomics is to facilitate the ability to engineer proteins, choosing either to inhibit or enhance specific, structure-based functions. Applications like x-ray crystallography, single-molecule fluorescence, and two-dimensional gel electrophoresis further this objective by providing a wealth of complementary information about protein function and structure.
About the Author
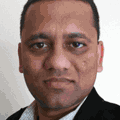
Ravi Guntupalli
Product Manager
Ravi Guntupalli is a product manager at Princeton Instruments, a leading provider of scientific grade CCD, ICCD, EMCCD and InGaAs based cameras and spectrographs for ultra low light imaging and spectroscopy applications. With over 14 years of experience in the field of low light imaging technonologies, Ravi has been working with researchers worldwide who are applying these technologies across life and physical sciences.
Manjul Shah
Applications Scientist, Teledyne Princeton Instruments
Manjul Shah is applications scientist at Teledyne Princeton Instruments (Trenton, NJ).
Mark Christenson
Life Sciences Business Manager, Roper Scientific
Mark Christenson is life sciences business manager at Roper Scientific (Tucson, AZ).
Jeff Grant
Senior Writer, Roper Scientific
Jeff Grant is senior writer at Roper Scientific (Tucson, AZ).