Quantum-cascade Lasers: Electrically pumped broadband random terahertz laser is highly directional
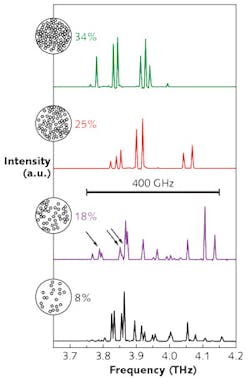
Commercial coherent terahertz-radiation sources have been around for more than a decade in the form of quantum-cascade lasers (QCLs), which are useful for both spectroscopy and imaging, often for security applications. One big disadvantage of the conventional terahertz-emitting QCL, however, is that because it is a subwavelength-confinement structure, it has a very divergent beam.
Researchers at Technische Universität Wien (TU Wien) and the Austrian Academy of Sciences (both in Vienna, Austria) have come up with a solution to the divergence problem by drawing from a laser concept that has often appeared in photonics research in the past few years—the random laser.
Random lasers typically have many scattering points distributed randomly or pseudorandomly in the laser cavity, which produce multiple laser modes that can loop from scatterer to scatterer within the cavity. While random lasers for the visible or near-UV tend to be based on polycrystalline scatterers, things are very different for the terahertz region, which has wavelengths three orders of magnitude longer. In fact, things are much easier: the researchers created scatterers simply by creating a random pattern of holes across an in-plane cavity to scatter light vertically out (see figure). The resulting device produces broadband emission (0.4 THz bandwidth for a center wavelength of about 3.9 THz) with an almost diffraction-limited narrow far-field output from the large cavity diameter.1
Differing hole-filling fractions
Such a laser can be modeled in a simplified way by assuming the randomly arranged holes are temporally coherent in-phase dipole emitters, each that have a given polarization direction. If all polarizations are aligned, the result is constructive interference that leads to a diffraction-limited beam. If all are maximally unaligned, deleterious interference effects are the result. In-between polarization alignments result in some, but not maximal, constructive interference.
Modeling the laser in more-sophisticated 3D and vectorial ways leads to an agreement with the experimental bandwidth of 400 GHz centered at 3.9 THz, and also shows that the cavity, although circular, does not support whispering-gallery modes because they are inhibited by the holes.
For the experiment, a 10-μm-thick planar electrically pumped semiconductor structure in a circular shape serves as the cavity. The researchers take advantage of the fact that because QCLs have a unipolar operation (only electrons, rather than electron-hole pairs), the cavity can be shaped almost arbitrarily without affecting electrical operation of the laser. So they went ahead and punched 20-μm-diameter holes through the cavity in random patterns. Different versions were made, with hole-filling fractions of 34%, 25%, 18%, and 8%, respectively.
The heat sinks for the devices were cryogenically cooled to 5 K, although the devices themselves reached temperatures of up to 130 K. The peak output powers in the vertical direction reached 40 mW for the more densely filled hole patterns, and less for the lower-density patterns. The divergence angle of the laser emission was about 7° full width at half-maximum (FWHM) for the laser with an 18% filling fraction. Because this is close to diffraction-limited, it implies that most of the emissions from the holes in the cavity surface were in phase with each other.
The electrically pumped nature of this random QCL is, of course, an advantage for its future possible use as a low-divergence terahertz light source.
REFERENCE
1. S. Schönhuber et al., Optica (2016); http://dx.doi.org/10.1364/optica.3.001035.
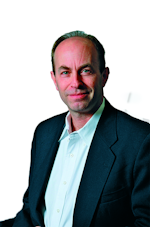
John Wallace | Senior Technical Editor (1998-2022)
John Wallace was with Laser Focus World for nearly 25 years, retiring in late June 2022. He obtained a bachelor's degree in mechanical engineering and physics at Rutgers University and a master's in optical engineering at the University of Rochester. Before becoming an editor, John worked as an engineer at RCA, Exxon, Eastman Kodak, and GCA Corporation.