PHOTONIC FRONTIERS: 50 YEARS OF LASERS: How laser output spread across the spectrum
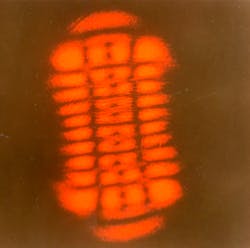
In the early days of automotive mass production, Henry Ford said customers could buy cars in any color they wanted, as long as it was black. In the first years of the laser age, users seeking visible lasers could get any color they wanted, as long as it was red. Although laser lines soon proliferated in the laboratory, very few became commercially viable, and for many years laser users had only a few choices.
We've come a long way since then. The range of wavelengths now spreads from quantum cascade lasers in the terahertz band to free-electron lasers in the x-ray band. New lasers have filled in the voids in the spectrum, and some of them are tunable. Harmonic generation, Raman shifting, and frequency mixing can shift laser output to new wavelengths. Nonlinear optics can spread laser light across a range of wavelengths to form a supercontinuum. You can't get everything you might want, but wander through a major laser show and you'll find an impressive range of laser sources, many not available a decade ago.
Fixed-wavelength lasers
The first generation of lasers emitted on fixed-wavelength transitions between pairs of well-defined energy levels. Theodore Maiman's ruby laser was a landmark not merely because it was the first laser but also because its deep red output was easily visible to anyone with normal color vision, making experiments easy. The first helium-neon (HeNe) lasers emitted at 1153 nm in the near-infrared (near-IR), but HeNe devices didn't take off until 1962, when Alan White and Dane Rigden of Bell Labs (Murray Hill, NJ) developed the 632.8 nm red version (see Fig. 1). The visible red HeNe quickly became the standard laser for research and measurement applications from holography to aligning sewer pipes.
Other fixed-wavelength lasers followed, scattered from the IR to the ultraviolet, but few were widely used. Neodymium (Nd) lasers became the standard near-IR sources at 1.06 µm, with carbon dioxide at 10.6 µm. Argon, krypton, and helium-cadmium offered visible output at higher powers and shorter wavelengths than helium neon, but at higher prices and lower efficiency. Nitrogen emitting at 337 nm became the standard ultraviolet laser until rare-gas-halide lasers displaced it in the late 1970s.
Fixed-wavelength lasers achieved the high intensities needed to produce nonlinear effects that changed the laser wavelength. By shining a ruby beam through crystalline quartz, Peter Franken at the University of Michigan (Ann Arbor, MI) was the first to generate the 347 nm second harmonic of ruby in 1961.1 Gisela Eckhardt and colleagues at Hughes Research Labs observed stimulated Raman scattering in 1962 when they illuminated nitrobenzene and other liquids with ruby pulses.2 Shorter pulses and higher peak powers produced more dramatic effects. In 1970, Robert Alfano and Sidney Shapiro at GTE Laboratories (then in Bayside, NY) reported that a combination of nonlinear effects produced a broadband supercontinuum, which they observed spanning the visible spectrum from 400 to 700 nm. 3
Tunable lasers
The first tuning of lasers was accomplished by applying magnetic fields to shift energy levels and thus the wavelengths of fixed-wavelength lasers. Joe Giordmaine and Robert C. Miller at Bell Labs (Murray Hill, NJ) later demonstrated optical parametric oscillation in lithium niobate.4 But broadly tunable laser output had to wait for the organic dye laser.
Peter Sorokin and John Lankard discovered the dye laser at the IBM Watson Research Center (Yorktown Heights, NY) in 1966 after they saw strong emission when they fired ruby pulses into a solution of chlor-aluminum phthalocyanine dye. Putting a pair of mirrors around the dye cell generated emission so intense it burned the emulsion on their film.5 In 1967, Bernard Soffer and B.B. McFarland at Hughes Research Labs (Malibu, CA) took the logical next step of making a tunable dye laser, replacing one cavity mirror with a diffraction grating that they turned to select which wavelength would oscillate in the cavity.6 In 1970, Benjamin Snavely's group at Eastman Kodak (Rochester, NY) used an argon-ion laser to pump the first continuous-wave dye laser.7
Able to deliver much more power in a narrow spectral band than conventional monochromators, tunable dye lasers became powerful research tools. They produced a series of spectroscopic breakthroughs in the 1970s, including work that earned Arthur Schawlow the 1981 Nobel Prize in physics. Yet dye lasers were complex devices with many practical drawbacks, including limited dye lifetimes and spectral ranges.
The next big step in tunable laser development was the solid-state vibronic lasers, in which the light-emitting ion changed vibrational and electronic energy levels simultaneously. Titanium-doped sapphire, developed by Peter Moulton at the MIT Lincoln Laboratory (Lexington, MA), has a fundamental tuning range of 675 to 1100 nm, much broader than any individual dye (see Fig. 2).8 Ti:sapphire also can be frequency-doubled to shorter wavelengths.Semiconductor diode lasers can't match the gain bandwidth of Ti:sapphire, but they can be made tunable in monolithic cavities. A pair of distributed Bragg reflectors with different periods serve as cavity mirrors in the sampled-grating distributed Bragg reflector laser invented by Larry Coldren at the University of California at Santa Barbara.9 Changing current or temperature alters the grating resonances to tune the laser. Software in fiberoptic transmitters sets the tunable laser to emit at a fixed wavelength.
Tailoring semiconductor laser wavelengths
Semiconductor laser wavelengths depend on the bandgap of the material in the active layer. In early semiconductor diode lasers these were set by the nature of the binary III-V compound, but improvements in the technology make it possible to tailor the wavelength by adjusting the semiconductor composition and structure.
The first step was development of ternary compounds such as gallium aluminum arsenide (GaAlAs), which could be lattice-matched to a GaAs substrate and emitted at shorter wavelengths as aluminum concentration increased. However, GaAlAs is unusual in its good match to the GaAs lattice, so the crucial step was to quaternary compounds such as InGaAsP, which gives a second degree of freedom to match lattice spacing as well as desired wavelength. J. Jim Hsieh made that step at MIT Lincoln Lab, and reported room-temperature operation of InGaAsP lasers emitting at 1.25 µm in 1977.10 Lasers with slightly different InGaAsP compositions, also on InP, soon followed for the low-loss fiber windows at 1.3 and 1.55 µm. Balancing the compositions of four elements covers an important range of wavelengths (see Fig. 3).Refinements in composition of GaAs-substrate lasers extended their output range to red wavelengths as short as 620 nm using AlGaInP. Then in 1996 Shuji Nakamura jumped to the blue end of the spectrum by making diode lasers from gallium indium nitride (GaInN) at Nichia Corp. (Tokushima, Japan).11 Blue diode lasers are now standard products, but green diodes have remained elusive. Now at UC Santa Barbara, Nakamura told Photonics West in January that he and colleagues at startup Kaai Inc. (Santa Barbara, CA) have developed 523 nm InGaN diodes to fill that hole in the diode-laser spectrum.
Optical pumping allows semiconductor lasers to reach additional wavelengths by avoiding the need to pass high current densities through the semiconductor. That relaxes design constraints, so quantum well structures can lase at otherwise unobtainable wavelengths. A pump diode illuminates the surface of a thin semiconductor disk with quantum-well layers near its surface, and the quantum wells emit light that resonates in an external cavity, producing an intense, high-quality beam. Called OPSLs (optically pumped semiconductor lasers) or VECSELs (vertical-external-cavity surface-emitting lasers), these devices have fundamental output in the near-IR, which can be frequency-doubled into the visible inside the laser cavity. Designs can be matched to specific wavelengths, such as the 441 nm line of helium-cadmium lasers, or the 577 nm yellow line for treating diabetic retinopathy.
Another novel structure is the quantum cascade laser, invented by Federico Capasso at Bell Labs in 1994, in which electrons pass through a stack of quantum wells in a biased semiconductor.12 In each quantum well, the electron drops to a lower sublevel of the conduction band and emits a photon with wavelength that depends on the structure and composition of the laser. Quantum cascade lasers have given easy access to otherwise hard-to-reach parts of the mid- and far-infrared and can be used to generate terahertz frequencies.
Other sources and outlook
Today's catalog of laser sources offers a rich variety of wavelengths that was hard to imagine in the first decades of the laser era. Moreover, nonlinear optics give us powerful tools to generate new wavelengths. High-order harmonics of high-power femtosecond pulses can generate coherent light deep into the extreme ultraviolet. Nonlinear processes can generate supercontinuums an octave or more wide, and femtosecond frequency combs, a Nobel-winning innovation that can measure optical frequencies with amazing precision.
Now we can even generate stimulated emission from free electrons, in the free-electron laser that John Madey invented in the 1970s. The FEL principle can be used from the infrared all the way to x-rays. Today the SLAC Coherent Light Source (Stanford, CA) is producing coherent x-rays at wavelengths from 0.15 to 1.5 nm.
Laser wavelengths have come a long way, and we now have a tremendous range of laser tools for a wide range of applications. Yet plenty of challenges remain for the next half-century.
REFERENCES
- P.A. Franken et al, "Generation of optical harmonics," Phys. Rev. Lett. 7, p. 118 (Aug. 15, 1961).
- G. Eckhardt et al, "Stimulated Raman scattering from organic liquids," Phys. Rev. Lett. 9, p. 455 (Dec. 1, 1962).
- R.R. Alfano and S.L. Shapiro, Phys. Rev. Lett. 24, p. 584 and 592 (Mar. 16, 1970); R.R. Alfano and S.L. Shapiro, Phys. Rev. Lett. 24, pp. 1217–1220 (June 1, 1970).
- J.A. Giordmaine and R.C. Miller, "Optical parametric oscillation in the visible spectrum," Appl. Phys. Lett. 9, pp. 298–300 (1966).
- P.P. Sorokin and J.R. Lankard, IBM Journal of Research and Development 10, p. 162 (1966).
- B.H. Soffer and B.B. McFarland, "Continuously tunable narrow-band organic dye lasers," Appl. Phys. Lett. 10, p. 266 (1967).
- O.G. Peterson et al., "CW operation of an organic dye solution laser," Appl. Phys. Lett. 17, 6, p. 245 (1970).
- P.F. Moulton, "Spectroscopic and laser characteristics of Ti:Al2O3," JOSA B 3, pp. 125–133 (January 1986).
- S. Lee et al, "Integration of semiconductor laser amplifiers with sampled grating tunable lasers for WDM applications," IEEE Quantum Electron. 33, pp. 615–627 (1997).
- J.H. Hsieh and C.C. Shen, "Room-temperature CW operation of buried-stripe double-heterostructure GaInAsP/InP diode lasers," Appl. Phys. Lett. 30, p. 429 (Apr. 15, 1977).
- S. Nakamura et al., "InGaN-based multi-quantum-well-structure laser diodes," Japan J. Appl. Phys. 2, 35(1B):L74-1 (1996).
- J. Faist et al., "Quantum Cascade Laser," Science 264 (5158), pp. 553–556 (April 1994).
Ted Maiman's achievement
May 16, 2010 marks the 50th anniversary of the birth of the laser in Theodore Maiman's lab at Hughes Research Laboratories (Malibu, CA). His ruby laser both experimentally realized previous proposals and opened entirely new doors.Earlier proposals envisioned the laser as a continuous optical oscillator, with low gain and relatively modest power. The leading candidates were four-level systems, with the laser transition on a pair of energy levels between the initial excited state and the final ground state. By the end of 1960, four-level lasers would be demonstrated in both solids (uranium-doped calcium fluoride) and gases (helium-neon).
Maiman's ruby laser was instead a three-level system, in which light excited chromium atoms to a high energy level, they dropped to a metastable state, then emitted stimulated emission when dropping to the ground state. The conventional wisdom was that ruby wouldn't work because its fluorescence efficiency was low and a population inversion was too difficult to produce. Yet when Maiman measured ruby on his own, he found its fluorescence efficiency actually was very high, holding out hope for a laser.
He then applied his own engineering ingenuity to design a ruby laser pumped by pulses from a photographic flashlamp that briefly excited most chromium atoms out of the ground state, creating the needed population inversion. When the pulse power from the flashlamp exceeded laser threshold, he observed the narrowing of ruby emission lines that had been expected during laser oscillation.
But he also produced something others had not predicted: a high-gain laser that produced bright pulses during the brief interval when the flashlamp inverted the population of chromium atoms in his little ruby rod. Moreover, Maiman's laser was easy to reproduce; within weeks TRG Inc., Bell Labs, and others had their own ruby lasers running, and were measuring pulse power in gillettes–the number of razor blades that the laser could penetrate.
Maiman's invention may look blindingly obvious with 20-20 hindsight, but that's a mark of his genius. Like all inventors, he extended earlier work, including his own development of a compact ruby microwave maser. He built his success on a solid understanding of ruby and maser physics, and an engineering expertise that made his ruby laser look deceptively simple. Once Maiman had marked the trail, others could follow, joining him in crossing the laser threshold.
About the Author
Jeff Hecht
Contributing Editor
Jeff Hecht is a regular contributing editor to Laser Focus World and has been covering the laser industry for 35 years. A prolific book author, Jeff's published works include “Understanding Fiber Optics,” “Understanding Lasers,” “The Laser Guidebook,” and “Beam Weapons: The Next Arms Race.” He also has written books on the histories of lasers and fiber optics, including “City of Light: The Story of Fiber Optics,” and “Beam: The Race to Make the Laser.” Find out more at jeffhecht.com.