PHOTONIC FRONTIERS: MATERIALS FOR SOLID-STATE LASERS: New materials expand capabilities of solid-state lasers
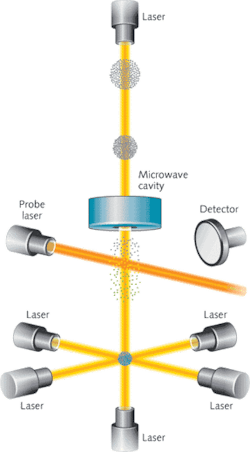
Although pump sources and cavity designs have evolved over the years, the raw materials for solid-state lasers have been slow to change. Solid-state physics is a complex field, and it has long been cheaper and easier to refine established laser materials such as glass, YAG, and YLF, than to develop new materials for commercial use.
That situation is changing, however, as decades of basic research are producing a better understanding of laser materials. New solid-state lasers are crossing the threshold of practicality, including semiconductors that are optically pumped rather than powered electrically. Three of the hottest new classes of materials are challenging the old standards: ceramic laser hosts, II-VI crystals such as zinc selenide doped with transition metals, and optically pumped semiconductor lasers (OPSLs).
Ceramic lasers
Ceramics are polycrystalline, made by fusing many small, randomly oriented grains. Familiar household ceramics are opaque or translucent because they are laden with internal flaws that scatter light. But with care, grains can be fused together cleanly enough to eliminate most scattering flaws, leaving the ceramic transparent enough for optical applications.
Tests of ceramic laser materials began in the 1960s but got little attention until 1995, when Akio Ikesue of World Lab (Nagoya, Japan) reported a ceramic neodymium-YAG laser with performance comparable to that of single-crystal material, stimulating a still-growing wave of ceramic-laser research.1
Advantages of ceramics include the ability to make them in much larger sizes than single crystals grown by conventional melt-solidification. Ceramics can be doped more heavily with the laser species, fabricated into fiber form, and made with internal structures that can’t be produced by single-crystal growth. Developers expect ceramic production to be cheaper than conventional crystal growth when fully developed. And sintering of some fine-grained ceramics in contact with a seed crystal can convert them into single crystals by a process called solid-state crystal growth.
Much of the pioneering work has been done in Nd:YAG. Developers say that the polycrystalline ceramic has better thermo-optical and optomechanical properties than conventional single-crystal YAG. Ceramic Nd:YAG is looking particularly good for very high powers. The Lawrence Livermore National Laboratory (Livermore, CA) used ceramic Nd:YAG amplifiers to generate a record average power of 67 kW from its Solid State Heat Capacity Laser (see Fig. 1).2 In September, a National Research Council review panel, called ceramic slab lasers “the most promising near-term technical approach for solid-state lasers” usable as weapons to counter rockets, artillery, and mortars.3
Ceramics can be produced from materials that can’t be grown by conventional single-crystal techniques, particularly the family of “sesquioxides” such as Lu2O3, Sc2O3, and Y2O3, which can be doped with rare-earth laser species and have melting points above 2400°C. Their high thermal conductivity and broad bandwidth make ceramics promising for high-power and ultrashort-pulse lasers, Ikesue wrote in a December review paper in Nature Photonics.4 Optical-pumping slope efficiency has reached 86% in Yb-doped Lu2O3.5
The technology of ceramic lasers is still developing, Ikesue wrote, but they have already attained “laser properties that cannot be achieved by conventional single-crystal technology.”
Transition-metal doped II-VI mid-IR Lasers
The mid-1990s also saw the first demonstrations of mid-infrared lasers based on wide-bandgap II-VI chalcogenides doped with transition metals such as chromium and iron.6 The spectroscopic and laser properties of these materials “are close mid-infrared analogs of titanium-doped sapphire,” making them particularly attractive for laser development, Sergey Mirov, Vladimir Fedorov, and Igor Moskalev of the University of Alabama at Birmingham (Birmingham, AL) wrote in a recent review paper.7
Early work focused on zinc selenide (ZnSe), which is well-developed, inexpensive, and transparent across a broad range of wavelengths. The most developed material in the family is Cr+2:ZnSe, which can be diode-pumped and oscillates from 1.9 to 3.1 µm. Continuous-wave operation is possible across most of that range, and optical conversion efficiency can exceed 60%. By optimizing material quality and making thin slabs of the gain material to improve thermal properties, the Alabama group, together with Photonics Innovations (Birmingham, AL) and the Air Force Research Laboratory (Dayton, OH) recently raised continuous-wave output to 13 W, limited by the available pump power and thermal management.
Zinc sulfide has attractive properties, including better thermal conductivity and resistance to optical damage than ZnSe, but the technology of doping ZnS is less mature. “We believe this material has a good future,” says Mirov. His group has succeeded in chromium-doping ZnS, obtaining more than 10 W from a Cr-doped ZnS laser in a nonselective cavity, and tuning output of more than 7 W across the range of 1.95 to 2.85 µm. Another promising material is iron-doped ZnSe. Room-temperature lasing has already been demonstrated in Fe+2:ZnSe, and Mirov is optimistic about prospects for room-temperature operation between 3.7 and 5.1 µm with broader tunability than quantum-cascade lasers, now the best tunable lasers available in that range. His group also has demonstrated lasing in optically pumped doped quantum dots of the same materials.Power levels can be improved, says Mirov. “We are talking of getting to hundreds of watts and even higher.” He also is looking to new applications, such as taking advantage of the ability of Cr-doped chalcogenide lasers to tune in and out of strong water absorption bands to make laser scalpels able to change their penetration depth into tissue.
Optically pumped semiconductors
Electrical pumping may eventually be possible for the doped II-VI compounds, but it isn’t desirable for other OPSLs, initially developed in the 1990s.8 These devices also are called VECSELs (vertical-external-cavity surface-emitting lasers), which highlights their kinship to VCSELs.
Mark Kuznetsov and Aram Mooradian originated the design to avoid the power limits imposed on electrically excited VCSELs by the complex internal structures needed to control current flow. The optically pumped version retains the Bragg reflector that serves as the rear cavity mirror in a VCSEL and quantum wells in the active region that emit light (see Fig. 2). However, the laser emission is not powered by recombination of carriers at a diode junction but by a pump diode that illuminates the disk surface. The OPSL uses an external cavity, with an output mirror separate from the semiconductor, leaving room for intracavity optics such as a harmonic generator.
Optical pumping can excite a broader area than electrical recombination, producing a larger emitting area that offers both better beam quality and higher power. Another big advantage is the ability to engineer the gain medium to give the desired absorption band and emission wavelength, says John-Mark Hopkins at the University of Strathclyde, Institute of Photonics (Glasgow, Scotland). Pumping is easy with photons having energy above the bandgap in the chip.Coherent (Santa Clara, CA) commercialized the technology and last year received the PhAST/Laser Focus World Innovation Award for its OPSL that emits 3 W at 577 nm in the yellow, matching the absorption peak of oxygenated hemoglobin (see www.laserfocusworld.com/articles/330753). Generating that exact wavelength concentrates laser energy in the retinal tissue affected by diabetic retinopathy and wet-form macular degeneration, a significant medical advance. It’s produced by frequency-doubling the beam from an OPSL emitting at 1154 nm.
Both flexible and powerful, the optically pumped design has been used with a wide range of III-V semiconductors, with fundamental output from 640 nm in the red to 2.3 µm in the infrared. Power levels can reach tens of watts on the fundamental and about 20 W on the second harmonic (see Fig. 3). The lasers are simple and compact and can be designed to emit at wavelengths needed for specific applications, including medical procedures and as replacements for larger and more expensive argon lasers emitting at 488 nm.
Hopkins says the field is advancing rapidly. Third- and fourth-harmonic generation is pushing deeper into the ultraviolet for potential use in small photolithography systems. He is developing sources with fundamental output at 1.9 to 2.8 µm as part of a European initiative, and has demonstrated gallium indium arsenide antimonide (GaInAsSb) chips pumped by 980 nm diodes that emit at wavelengths to 2.3 µm.9 Potential applications include sensing and free-space communications at eye-safe wavelengths.
Outlook
The old solid-state warhorses like neodymium and ytterbium are far from becoming endangered species. Their solid performance and established technology have earned them their niche. But with advances in ceramic lasers, they may come in an increasing variety of forms and at higher powers.
They will also have plenty of competition from new materials emitting at other wavelengths. Doped chalcogenide lasers are pushing further into the infrared region beyond 2 µm where few lasers have been available for such promising applications as sensing in surgery. And optically pumped semiconductor lasers have proved impressively adaptable at generating watt-level powers from the ultraviolet well into the infrared. “If you’ve got specific applications, we can now tailor the laser to meet the application requirements,” says Hopkins. That’s good news for laser users.
REFERENCES
- A. Ikesue et al., J. American Ceramic Society 78, p. 1033 (1995).
- A. Heller, Science & Technology Rev., April 2006, p 10.
- National Research Council, Committee on Directed Energy Technology for Countering Indirect Weapons, Review of Directed Energy Technology for Countering Rockets, Artillery, and Mortars (RAM) (National Academies Press, 2008).
- A. Ikesue and Y.L. Aung, Nature Photonics 2, p. 721 (December 2008).
- R. Peters et al., CLEO 08, paper CTuKK4.
- L.D. DeLoach, et al., IEEE J. Quantum Electron. 32, p. 885 (Jun. 1996).
- Se. Mirov et al., IEEE J. Selected Topics In Quantum Electron. 13(3) p. 810 (May/June 2007).
- M. Kuznetsov, F. Hakimi, R. Sprague, and A. Mooradian, IEEE J. Select. Top. Quantum Electron. 5, p. 561 (1999).
- J.M. Hopkins et al., Optics Express. 15, p. 8212 (2007).
About the Author
Jeff Hecht
Contributing Editor
Jeff Hecht is a regular contributing editor to Laser Focus World and has been covering the laser industry for 35 years. A prolific book author, Jeff's published works include “Understanding Fiber Optics,” “Understanding Lasers,” “The Laser Guidebook,” and “Beam Weapons: The Next Arms Race.” He also has written books on the histories of lasers and fiber optics, including “City of Light: The Story of Fiber Optics,” and “Beam: The Race to Make the Laser.” Find out more at jeffhecht.com.