PHOTONIC FRONTIERS: LASER FILAMENTATION - Femtosecond pulses create self-guiding filaments
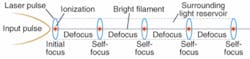
Tightly focused laser pulses have long been known to ionize air, and it is now possible to produce extreme conditions by concentrating beams in time and space to intensities exceeding 1018 W/cm2. However, focusing purely on achieving the highest possible peak power overlooks some important effects that occur only at somewhat lower power levels, such as laser filamentation. First observed a dozen years ago in a key experiment, filamentation occurs when two nonlinear effects offset each other to trap ultrashort optical pulses in a self-confined filament stretching long distances in air.
Similar effects have been observed in transparent solids and liquids, and subsequent experiments have stretched filament propagation to kilometers in air. In the process, the femtosecond pulses are squeezed to even shorter durations, and stretched into a supercontinuum spanning the near-UV to the near-IR. Developers are looking at a range of potential applications including remote sensing, nonlinear spectroscopy, and guiding electrical discharges through air.
A balance of effects
The goal of the initial experiments performed by Gerard Mourou’s group at the University of Michigan (Ann Arbor) was to study the propagation in air of 200 fs pulses with 10 GW peak power for potential pulsed lidars. The researchers expected the optical Kerr effect to produce self-focusing of the beam arising from the second-order dependence of refractive index n on beam intensity I:The nonlinear term increases the refractive index as beam intensity increases, causing self-focusing that bends the sides of the beam toward its center. However, the nonlinear term n is small for air, so high powers are needed to trigger self-focusing.
The Michigan experiments produced something unexpected because the light in the center of the laser beam was focused to an intensity high enough to ionize air molecules. The resulting plasma had a refractive index lower than that of neutral air, so it defocused the beam. This defocusing offset the self-focusing effect, reducing the beam intensity so ionization stopped and self-focusing again dominated. As a result, the femtosecond pulses are alternately self-focused and defocused, self-guiding the light on a straight path (see Fig. 1).1 The alternating self-focusing and defocusing also “clamps” the intensity of the beam, limiting peak power in a self-focusing region to on the order of 5 × 1013 W/cm2.2 Some filaments have propagated through several kilometers of air.3
Short pulses are also essential to producing filaments. Although multiphoton ionization is needed to produce the defocusing plasma that helps create the self-guiding effect, it is also important not to accelerate the electrons to energies high enough for them to ionize other gas molecules. If electrons cross that energy threshold, they can trigger a cascade of ionization, forming a strongly absorbing plasma that can block the beam. Femtosecond pulses are so short that their electric fields drop back to zero before they can accelerate electrons to high velocities. However, pulses lasting tens of picoseconds or more can accelerate electrons to produce gas breakdown, so filamentation couldn’t be observed until femtosecond lasers were developed, according to See Liang Chin of the University of Laval (Quebec, Canada).
The visible part of the filament is essentially a trace of the path followed by the pulses. Molecules ionized by the peak-intensity light at the center of the pulse fluoresce, forming a line only about 100 µm wide. The self-guided light is spread through a larger volume several hundred microns wide that surrounds the visible filament. That broad distribution of light energy makes filaments surprisingly robust and self-healing. They redistribute guided light energy from the broad region around the filament if they hit an obstacle-even an opaque 100 µm particle as big as the visible filament itself.2 Powerful pulses can form multiple filaments, which move in parallel, but can recombine when their individual intensity drops toward the minimum threshold needed to sustain a filament.
Pulse compression and supercontinuum generation
The femtosecond pulse is also affected by the nonlinear interactions between the light and the transmission medium, which can be a transparent solid or liquid, as well as air.
One result is pulse compression. Pulses initially lasting tens to a few hundred femtoseconds are squeezed down to several femtoseconds. The best results to date are pulses lasting less than 5 fs, about two optical cycles.5 In theory, pulses can be squeezed to almost a single cycle of light. If you could see the pulse itself, it would be a tiny disk of light on the order of a micron thick and perhaps 100 µm wide, moving at the speed of light in a direction perpendicular to the surface of the disk. Successive pulses, sometimes called “bullets” of light, are separated by the laser’s repetition rate.The forces that compress the pulse in time broaden its spectral bandwidth. Self-phase modulation makes the trailing end of the pulse increasingly steep, spreading the laser pulse to shorter wavelengths, so an initial pulse from a Ti:sapphire laser centered at 800 nm winds up spread across the entire visible spectrum to wavelengths as short as 300 nm (see Fig. 2). The pulse also spreads to longer wavelengths, and infrared emission has been observed all the way to 5 µm, but the intensity is much lower than at shorter wavelengths. It is possible to convert much of the initial energy of the femtosecond pulses into a continuum, sometimes called a “white-light laser.” The intensity is high enough to generate third-harmonic emission at wavelengths to 230 nm.
Self-phase modulation also causes a wavelength gradient in the cone of light emitted at small angles to the filament, creating a rainbow array of colors (see Fig. 3). Shorter wavelengths are emitted at larger angles to the filament. The rings appear to be discrete, but the dark zones actually are fringes produced by interference of light from closely spaced filaments.6Applications
The ability to generate broadband continuum pulses opens new opportunities in remote sensing. Conventional lidars can remotely measure concentrations of atmospheric trace gases by monitoring backscattering from a laser beam as a function of time, but with a monochromatic beam they are limited to one gas at a time. The broadband emission from femtosecond filaments spans a broad range of the spectrum, so time-resolved measurements of the backscattered spectrum can monitor absorption bands of many important gases between 230 nm and 4 µm, including methane, ozone, water vapor, and nitrogen dioxide. The emission range includes 3 to 3.5 µm absorption bands of volatile organic compounds, which conventional differential absorption lidar can’t measure properly because their absorption bands overlap. The broadband pulse also can stimulate fluorescence from many species; Chin says remote detection of chemical or biological warfare agents in air is feasible with a single femtosecond laser.
Femtosecond filaments can also deliver extremely high light intensity to remote sites, causing breakdown of the target to study its composition.7 Called remote filament-induced breakdown spectroscopy, it is an extension of laser-induced breakdown spectroscopy, which detects materials by analyzing the spectrum of a laser-produced plasma. The nanosecond pulses previously used can propagate only over short distances, but femtosecond filaments can span much longer distances. Recently it has been used to detect salt-water aerosols at parts-per-million levels at distances to 70 m, and developers say it could be stretched to the kilometer range.8
Another intriguing possibility is controlling lightning strikes by directing the current along the ionized channel. Laser lightning rods were first proposed in the 1970s, but were impractical then because nanosecond pulses could not sustain ionized channels. Femtosecond filaments can do much better, guiding high-voltage discharges up to 4.5 m.9
Outlook
Femtosecond filaments are still in their early days. Berge notes that more work is needed on filament propagation in solids and liquids, where chromatic dispersion and nonlinear absorption play important roles. More work is also needed to develop more compact and easier-to-use femtosecond lasers; the 5 TW French-German “Teramobile” laser used in much early research occupies a small trailer.
But the potential rewards are large. Self-guiding femtosecond filaments open new opportunities, including production of high-order harmonics. In the longer term, they could lead to dramatic breakthroughs, such as power beaming through plasma channels and perhaps new types of propulsion.
REFERENCES
(* indicates comprehensive review papers)
1. A. Braun et al., Optics Lett. 20, 73 (1995).
2. S.L. Chin, Physics in Canada 60, 273 (Sept.-Oct. 2004).
3. *A. Couairon and A. Mysyrowicz, Physics Reports 44, 47(2007).
4. *L. Berge, S. Skupin, and R. Nuter, J. Kasparian, J.-P. Wolf, arXiv:physics/0612063 (Dec. 6, 2006).
5. A. Guandalini et al, paper CFO1, CLEO 2007.
6. *S.L. Chin et al, Can. J. Phys. 83, 863 (2005).
7. K. Stelmaszczyk et al., Appl. Phys. Lett.85(18) 3977 (Nov. 1, 2004)
8. J.-F. Daigle et al., paper CThY5, CLEO 2007.
9. R. Ackermann, et al., Appl. Phys. B: Lasers & Optics82, 561 (2006).
10. J. Kasparian et al., Optics Lett. 25, 1397 (Sept. 15, 2000)
About the Author
Jeff Hecht
Contributing Editor
Jeff Hecht is a regular contributing editor to Laser Focus World and has been covering the laser industry for 35 years. A prolific book author, Jeff's published works include “Understanding Fiber Optics,” “Understanding Lasers,” “The Laser Guidebook,” and “Beam Weapons: The Next Arms Race.” He also has written books on the histories of lasers and fiber optics, including “City of Light: The Story of Fiber Optics,” and “Beam: The Race to Make the Laser.” Find out more at jeffhecht.com.