Photonic Frontiers: Laser Acceleration: Short pulses speed particles
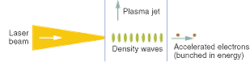
The concept of laser acceleration of charged particles dates back to 1979 when Toshi Tajima and John M. Dawson predicted that intense laser pulses could create a wake of plasma oscillations that could accelerate electrons to high energy.1 Their computer simulation of the effect attracted considerable interest because it held out the prospect of useful acceleration over much shorter distances than conventional particle accelerators. However, the short-pulse lasers of the day could not generate the peak powers needed for useful laser acceleration.
That changed with the development of chirped pulse amplification, which can generate extremely high peak powers in ultrashort pulses. The past several years have seen remarkable experimental progress, first with electrons and more recently with protons and heavier ions. Early experiments produced particles over a wide energy range, but recent results have narrowed the range of energies, a crucially important feature for applications that require precise control over particle energies.
Particle acceleration basics
Traditionally, charged particles have been accelerated by passing them through long metal tubes in which alternating electromagnetic fields were applied to a series of segments. The applied fields reverse as the particles pass through the segments, so the fields always accelerate the particles. The longer the tube, the more acceleration is applied to the particles, and the higher their energy. Laboratory-scale accelerators have meter-long tubes, but the accelerators used in cutting-edge high-energy physics can be several kilometers long. The maximum acceleration possible depends on the accelerator structure and the power of the alternating field. The upper limit is acceleration that increases energy by tens of megaelectronvolts per meter of tube length, so very high energies require huge accelerators.
Laser acceleration can generate much higher fields, so acceleration distance can be much shorter—typically a millimeter to drive electrons to 100 MeV. Firing powerful ultrashort pulses into a plasma or solid generates extremely intense electric fields, which can reach teravolts per meter at the instant of peak intensity. These fields overwhelm the electric attraction between the positive nucleus and the negative electrons, freeing both electrons and positive ions. The process also generates intense fields that accelerate the particles to high energies over short distances.
“Electron acceleration and proton acceleration are fundamentally different,” says Thomas Katsouleas of the University of Southern California (Los Angeles, CA), so different approaches have been developed for the two. The original laser-wake-field approach proposed by Tajima and Watson deposits energy in a plasma and works best for electrons. Protons and ions are much heavier and better accelerated by firing laser pulses that explode thin-film targets, freeing bursts of charged particles.
Laser-wake-field acceleration
Wake-field acceleration is often compared with surfing. When an intense laser pulse hits a plasma, it creates a density wave of free electrons. The electrons, in turn pull positive ions-protons or heavier nuclei-along with them, creating a density wave as they pass through the plasma (see Fig. 1). The density wave carries the free electrons with it, and the electrons can reach 100-MeV energies within a millimeter, roughly 1/5000th the distance needed in a conventional accelerator.
Like catching an ocean wave to surf, coupling a laser pulse into a plasma is tricky, and the process took time to perfect. The first experiments showed very fast acceleration, but it took time to increase the number of accelerated electrons and focus them in a narrow beam. In 2002, researchers accelerated a burst of 100 million electrons that spread within an angle of only three degrees, but their energy diverged widely.2 That was a concern. “For a lot of applications it’s the ‘holy grail’ to get a monoenergetic beam,” Katsouleas says.A major advance came in 2004, when three groups reported much narrower ranges of electron energy in papers that appeared in the same issue of Nature.3, 4, 5 The key to their success was finding ways to inject a clump of electrons into a small part of the plasma so the electrons can be accelerated collectively to nearly the same energy. The researchers fired pulses with peak powers of 10 to 30 TW and lengths of 30 to 55 fs into gas jets 2 mm long. By creating plasma channels or adjusting the laser beam to guide the density waves through the jets, they managed to constrain energy spread to no more than 24% for up to a few billion electrons (see Fig. 2).
Laser ion acceleration
Protons and positive ions are too heavy for the wake-field approach to accelerate them effectively. Instead, researchers blow them away by hitting a thin, dense foil with a pulse reaching higher than 1018 W/cm2. The electric field is so much stronger than the nuclear attraction so it blows electrons out the back of the exploding foil at relativistic speeds. The electric charge of the accelerating electrons pulls protons or heavier ions along behind, accelerating the positive ions over micrometer-scale distances. “This is a pure one-stage process,” says Juan Fernandez of the Los Alamos National Laboratory (Los Alamos, NM).
Exploding foils were long known from inertial-confinement fusion experiments. However, they were not seriously considered for laser acceleration until the Lawrence Livermore National Laboratory (Livermore, CA) unexpectedly generated a well-controlled proton beam by firing its Petawatt laser at gold foils.6 Analysis showed that the protons came from impurities in the foil. The beam was intense, but ion energy was distributed over a wide range, and many applications require monoenergetic beams. Medical therapy, for instance, requires uniform energy to ensure the particles all penetrate the same depth.
A pair of experiments reported in January in Nature took a big step toward that goal, using different approaches to generate beams of protons and carbon ions with limited ranges of energy.A team at Friedrich Schiller University (Jena, Germany) produced the proton beam by focusing 10-TW, 80-fs pulses from a Ti:sapphire laser to an intensity of 3 × 1019 W/cm2 on a 5-µm titanium foil. On the other side of the foil was an array of polymer dots 0.5 µm thick and 20 µm across. When a laser pulse hit the metal side of the foil behind a polymer dot, it blew off a cloud of hot electrons on the side of the dot, which in turn pulled protons from the polymer behind them (see Fig. 3). A plot of energy of the roughly 100 million laser-accelerated protons showed a narrow peak at 1.2 MeV, which was matched in simulations. The group calculates it could accelerate all 800 million protons in the polymer dot to an energy peak of 173 MeV—suitable for treating deep-seated tumors—if it had a laser that could deliver peak intensity of 1021 W/cm2.7
Fernandez’s group at Los Alamos concentrated on the more difficult problem of accelerating heavier ions. It focused 30‑TW, 600-fs pulses onto 10-µm spots on a 20-µm palladium foil with a thin graphite layer on the back. The intensity of 1019 W/cm2 blew relativistic electrons off the surface of the rear of the foil, which in turn accelerated highly ionized carbon atoms. The group found a 17% spread in the mean energy of about 36 MeV for the most abundant ions, C+5.8
A key advantage of the laser approach is the ability to generate much higher ion currents than can conventional accelerators. Mutual repulsion of ions limits current in a conventional accelerator, but the laser accelerator can produce multiple kiloampere pulses because it produces a neutral beam containing electrons as well as ions, says Fernandez. “You’re shooting a plasmoid” that doesn’t want to fall apart.
Outlook
Laser accelerators are not going to replace the gigantic particle accelerators used in particle physics research. In principle, wake-field accelerators might be extended to produce high-energy electrons—but not protons. “You’re never going to make very high-energy proton beams this way,” says Fernandez.
But laser acceleration has two other big strengths. Because they use very intense fields to accelerate particles over short distances, they can be made small enough to fit in a laboratory, especially as the size of high-power, short-pulse laser comes down. Laser accelerators can also produce higher-power beams. This combination makes them attractive for a wide range of applications. One is treating tumors with heavy ions, which deposit little energy until their velocity slows, zapping cancer cells deep inside the body without killing tissue along its path. Laser-accelerated beams might be used for fast ignition in inertial-confinement fusion. And once laser accelerators become available, more applications seem sure to appear.
REFERENCES
1. T. Tajima and J. M. Dawson, Phys. Rev. Lett. 43, 267 (July 23, 1979).
2. V. Malka et al., Science 298, 1996 (2002).
3. S.P.D. Mangles et al., Nature 431, 535 (Sept. 30, 2004).
4. C.G.R. Geddes et al., Nature 431, 538 (Sept. 30, 2004).
5. J. Faure et al., Nature 431, 541 (Sept. 30, 2004).
6. R. A. Snaveley et al., Phys. Rev. Lett. 85, 2945 (2000).
7. H. Schwoerer et al., Nature 439, 455 (Jan. 26, 2006).
8. B.M. Hegelich et al, Nature 439, 441 (Jan. 26, 2006).
About the Author
Jeff Hecht
Contributing Editor
Jeff Hecht is a regular contributing editor to Laser Focus World and has been covering the laser industry for 35 years. A prolific book author, Jeff's published works include “Understanding Fiber Optics,” “Understanding Lasers,” “The Laser Guidebook,” and “Beam Weapons: The Next Arms Race.” He also has written books on the histories of lasers and fiber optics, including “City of Light: The Story of Fiber Optics,” and “Beam: The Race to Make the Laser.” Find out more at jeffhecht.com.