PHOTONIC FRONTIERS: ATTOSECOND PHYSICS - Attosecond pulses open new window on atomic physics
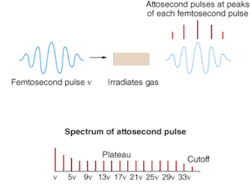
Strictly speaking, purely optical pulses cannot be shrunk to less than a femtosecond in length. The transform limit requires a spectral bandwidth broader than the visible spectrum to produce subfemtosecond pulses. However, optical femtosecond pulses can be used to generate high-harmonic pulses lasting attoseconds and spanning a much larger range of frequencies in the vacuum-ultraviolet and soft-x-ray regions.
These attosecond (as) pulses are opening the door to probing new physical processes. Femtosecond optical pulses made it possible to examine the process of molecular chemistry by slicing time into such small pieces that it froze the action. Similarly, attosecond physics offers a way to study the process of electronic transitions in atoms, and it’s become one of the hottest fields in optical research.
Nonlinear high-harmonic generation
The fundamental limit on optical pulse duration is set by the uncertainty principle, which holds that the product of uncertainty in photon energy and pulse duration must be above a minimum value,The leading approach is called high harmonic generation. Focusing an intense laser pulse into a jet of rare gases generates high-order harmonics at odd multiples of the frequency of the initial laser pulse (see Fig. 1). The lowest-order harmonics are most intense, but higher-order harmonics have similar intensities over a broad range of frequencies up to a cutoff value. Filtering out the lowest harmonics creates a broad frequency comb similar to the spectral modes of a modelocked laser, but reaching into the extreme-UV. Coherent superposition of these frequencies across a broad enough range can produce extremely narrow pulses. In principle, 100‑as pulses can be formed from a frequency comb of 5000 THz, equivalent to about 40 eV in energy.
Physically, the process depends on the rapid fluctuations of the electric field in powerful femtosecond pulses. When the light encounters atoms, a field that’s powerful can overwhelm the pull of the nucleus on valence electrons, which tunnel out of the atom. However, the field quickly reverses direction, pulling the escaping electron back toward the nucleus, and the recaptured electron releases its extra energy in a burst of radiation lasting a few hundred attoseconds.
The field of the laser pulse is so powerful that the affected electrons follow well-defined trajectories within the atom, which can be described by classical physics. The field actually pulls only part of the electron’s probability field out of the atom, which when released returns to collide with the rest of the electron’s probability field, like a sticky elastic blob. Because the electron collides with itself, the radiation from the collision is coherent, explains theorist Joseph Eberly of the University of Rochester, Institute of Optics (Rochester, NY). He says the electron radiates energy like a classic oscillating dipole, which emits at well-separated harmonic peaks rather than in a broad band.
Tricky technology
Experimentalists have developed sophisticated techniques to make attosecond pulses more useful. Normally the excited electron emits bursts every half-cycle of the pump light, so a single femtosecond optical pulse yields a series of attosecond bursts.Single attosecond pulses are more useful, and in 2001 Ferenc Krausz, then at the Technical University of Vienna, took another key step by producing the first single attosecond x-ray pulses, estimated at 650 as in duration (see Laser Focus World, February 2002, p. 17). The following year, his group used single attosecond pulses for time-resolved spectroscopy, but the pulses were not as well controlled as desired. In 2003, Krausz and Theodor Hänsch of the Max-Planck-Institute for Quantum Optics in Garching, Germany, developed a way to isolate single reproducible pulses. That required exacting control over the phase of the electric field within the envelope of the pulse amplitude, so the peak amplitude of the field occurred at exactly the moment the pulse amplitude reached its peak value, called a cosine pulse (see Fig. 2). Since then, Krausz has improved the technique for aligning the phase and amplitude by using the attosecond extreme-UV pulses to ionize atoms, then measuring how the field strength of the femtosecond visible pulses by how it changes the motion of the free electrons.
Measuring attosecond pulses poses its own challenges. High-precision techniques developed for femtosecond pulses rely on nonlinear optics. Attosecond pulses are not only faster, but span extreme-UV wavelengths where the nonlinear materials used in many femtosecond measurements strongly absorb light. So far, most groups demonstrating attosecond pulse generation have had to develop their own the techniques to verify their results.
Nonetheless, the technology is spreading, and new groups are developing their own experiments, largely in fundamental physics and metrology.
The allure of attoseconds
The allure of attosecond pulses is that they open a new frontier in time. Femtosecond pulses did the same in the 1980s, recording the fastest events ever captured, and showing processes never seen before. Ahmed Zewail of Caltech launched the field of femtochemistry by recording the progress of chemical reactions between molecules, revealing a previously unknown world and earning the 1999 Nobel Prize in Chemistry. Attosecond pulses can show the motion of electrons in atomic processes-and the precedent of Zewail’s Nobel is helping to encourage researchers’ natural curiosity.
Experimental physicists have led the rapid development of the field over the past several years. The first to report that high-harmonic generation could generate trains of subfemtosecond x-ray pulses was a group led by Dimitris Charalambidis at the University of Crete in Greece in 1999. Others soon followed, refining the technique and generating shorter and shorter pulses, but applications for repetitive pulse trains were limited, particularly at the low powers they produced. The developing of techniques for producing single, repeatable pulses opened the floodgates to serious experiments in attosecond physics. For instance, Krausz, now at the Max-Planck-Institute for Quantum Optics, has used attosecond pulses to observe the rearrangement of electrons in krypton atoms after removal of an inner-shell electron.Perhaps the most novel experiment so far was a version of the classic double-slit experiment performed at the Technical University of Vienna by Gerhard Paulus, who has joint appointments at Texas A&M University and Ludwig-Maximilians University in Munich, Germany. Instead of passing electrons through a pair of slits in space, he created slits in time using 5‑ns pulses from a Ti:sapphire laser. The pulses were timed to control the phase of the electric field in the amplitude envelope. In some, the pulses had a pair of positive maxima on either side of a central minimum; in others, a single maximum was in the center, with negative minima on either side. Both the primary peak and the secondary peaks had enough energy to eject some electrons from argon atoms in a gas cell, but they ejected the electrons in different directions. Paulus placed detectors at both sides, and found a set of interference fringes-in time-at the detector that monitored electrons ejected by the pair of pulses. The two peaks, separated by one wave interval, had interfered with each other in time (see Fig. 3).
Theoreticians are working to explain the growing body of experimental results. The strength of the laser field helps by simplifying interactions so the affected electrons move in a straight line, so “the physics become one-dimensional,” says Eberly. That simplification offers theoreticians a way to deal with multielectron interactions impossible to model in a full quantum-mechanical model, encouraging Eberly to tackle three-electron interactions.
The research has just begun. “We’re on the ground floor of the field,” says Anthony Starace of the University of Nebraska at Lincoln. “Where it’s going to lead is still not evident, but everyone feels there’s lots of possibility for new science.”
FURTHER READING
P. Agostini and L. F. DiMauro, Reports on Progress in Physics 67, 813 (2004).
H. Kapetyn, M. Murnane, and I. Christov, Physics Today 58, 39 (March 2005).
About the Author
Jeff Hecht
Contributing Editor
Jeff Hecht is a regular contributing editor to Laser Focus World and has been covering the laser industry for 35 years. A prolific book author, Jeff's published works include “Understanding Fiber Optics,” “Understanding Lasers,” “The Laser Guidebook,” and “Beam Weapons: The Next Arms Race.” He also has written books on the histories of lasers and fiber optics, including “City of Light: The Story of Fiber Optics,” and “Beam: The Race to Make the Laser.” Find out more at jeffhecht.com.